Biomimetic Strategies to Develop Bioactive Scaffolds for Myocardial Tissue Engineering
Abstract
The aim of this paper is to provide an overview of the results of the research activity carried out in our laboratories, over the last 10 years, in relation to the development of strategies for the production of biomimetic and bioactive scaffolds for myocardial tissue engineering. Biomimetic and bioactive polymeric scaffolds for cardiac regeneration were designed and manufactured in our laboratories and their morphological, physicochemical, mechanical and biological properties were investigated by different techniques, such as scanning electron microscopy, infrared chemical imaging, swelling test, in vitro degradation assessment, dynamic mechanical analysis, in vitro and in vivo biological tests. Biomimetic scaffolds, able to favor tissue regeneration by mimicking nature, were engineered by different strategies, comprising: (i) the imitation of the composition and interactions among components of the natural extracellular matrix (ECM), by mixing of proteins and polysaccharides; (ii) the material surface modification, using both traditional and innovative techniques, such as molecular imprinting; (iii) the incorporation and release of specific active agents and (iv) the production of scaffolds with a microarchitecture similar to that of native ECM. All the developed strategies were found to be effective in creating materials able to influence cellular behavior and therefore to favor the process of new tissue formation. In particular, the approach based on the combination of different strategies aimed at creating a system capable of communicating with the cells and promoting specific cellular responses, as the ECM does, has appeared particularly promising, in view to favor the formation of a tissue equivalent to the cardiac one.
1. INTRODUCTION
The goal of tissue engineering is to overcome the intrinsic limits of prosthesis and implants, aiming at the creation, repair or replacement of tissues and organs damaged or no longer able to function. A key step, within a tissue engineering process, is the design and manufacture of a scaffold capable of hosting the cells and favoring all the cellular processes that are necessary for the formation of a new tissue, which is equivalent to the natural one. Scaffolds are porous templates intended to support and stimulate the growth of new healthy tissues in three dimensions and then to safely dissolve, once they have performed their functions, thus leaving the body to remodel the tissue to its natural form. The rate and quality of tissue integration depend on the physicochemical, biological and structural properties of the scaffold, including size, distribution, and interconnectivity of the pores.
In recent years, it has been widely demonstrated that the ideal scaffold is the one that not only supports cell adhesion and growth but is also able to influence cell response by promoting all those processes that are essential for the formation of a new tissue, capable of replacing the natural one [1]. Through the use of different functionalization strategies, it is possible to develop scaffolds able to provide signals that can guide and promote specific cellular responses that are at the basis of tissue regeneration, similar to cell-extracellular matrix (ECM) interactions that occur during tissue development. These systems can be defined as biomimetic and bioactive scaffolds.
In this paper, we want to illustrate the different strategies and methods developed in our laboratories in recent years to create biomimetic polymeric scaffolds for the engineering of myocardial tissue.
The ECM represents the scaffold of native tissues, that the anchorage-dependent cells use to anchor themselves, in order to carry out all the activities that are necessary for the formation and maintenance of the tissue to which they belong. In the case of cardiac tissue, ECM serves as an anisotropic structural scaffold to guide aligned cellular distribution and organization. It accommodates contraction and relaxation of cardiomyocytes and facilitates force transduction, electrical conductance, intracellular communication, and metabolic exchange within the myocardial environment [2, 3].
Therefore, the first strategy that we used for the creation of biomimetic scaffolds, intended for applications in the cardiovascular area, is based on the imitation of the chemical composition of the natural ECM, trying to reproduce the interactions that specifically exist between protein and polysaccharide components of native ECM [1].
Another important consideration is concerning cell attachment to the scaffold surface. Cell adhesion represents the first fundamental event in the engineering of a tissue, therefore the modification of the scaffold surface to favor the adhesion event is another interesting method to create a biomimetic system. Surface modifications of 3D scaffolds were implemented through different procedures, including the binding of signaling peptides that are specifically recognized by receptors on cell surface. Peptides were anchored to the scaffold surface through chemical immobilization, thanks to the creation of covalent bonds between peptide groups and activated functional groups introduced on the scaffold surface [4]. Alternatively, the adsorption on the scaffold surface of specific adhesive proteins for subsequent promotion of desired cellular responses was achieved by an innovative application of Molecular Imprinting as a scaffold functionalization strategy, proposed for the first time by our research group [5-7].
We also produced bioactive scaffolds through loading with bioactive agents, such as recruitment or growth factors, whose release influences cellular processes that are essential for the formation of a new tissue. In particular, both injectable [8] and preformed 3D scaffolds [9] loaded with growth factors (GFs) were developed. Alternatively, scaffolds were functionalized by deposition of molecularly imprinted particles (MIP) with recognition properties towards a stem cell recruitment agent, in order to promote enrichment on the scaffold of this factor, stimulating the regeneration process [10].
Finally, microfabricated scaffolds mimicking the microarchitecture of cardiac ECM were produced by soft-lithography [11], as it has been shown that tissue architecture is strictly linked to tissue function, and therefore a scaffold with a structure capable of mimicking the native ECM could be extremely advantageous.
2. BIOMIMETIC SCAFFOLDS BY BLENDING NATURAL POLYMERS TO MIMIC ECM
An interesting strategy for the production of biomimetic scaffolds, for several tissue engineering applications, is based on the blending of proteins and polysaccharides, which aims to replicate the composition of the native ECM [12-20].
Systems based on alginate/gelatin (AG) blends, with a 20/80 weight ratio, were developed and characterized in our laboratories for potential use as cardiac ECM substitutes [1]. The 20/80 weight ratio between the two natural polymers was chosen after a preliminary screening work on AG blends with different weight ratios [21], being also the most similar to the weight ratio between the polysaccharide and protein components in native cardiac ECM. AG porous scaffolds in the form of sponges were prepared by a freeze-drying technique and subsequent ionic and chemical cross-linking procedures. The scaffolds were subjected to a complete morphological, physicochemical, functional, mechanical, and biological characterization, and their properties were compared with those of natural porcine myocardium [1] (Fig. 1a-c).
AG sponges showed a highly porous and homogeneous structure with well-interconnected pores and a surface porosity very similar to that of decellularized myocardium (Fig. 1a, and c) [1]. Their porosity resulted higher than that of native tissue, but this can be considered an advantage for promoting cell infiltration and colonization, thus favoring tissue regeneration.
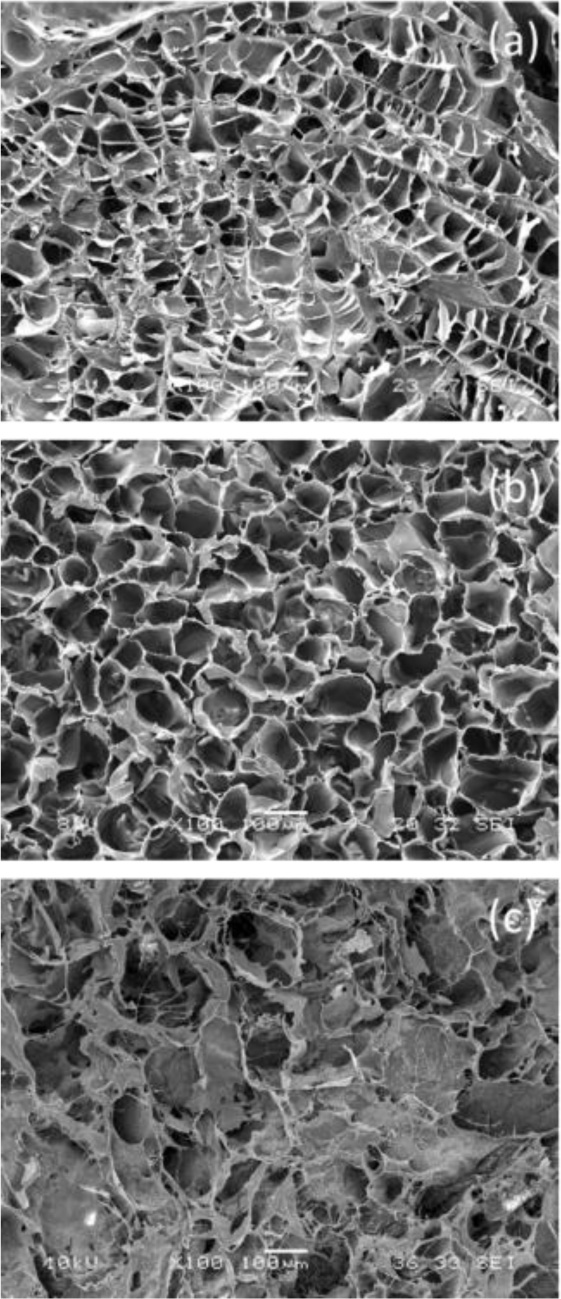
Infrared analysis showed that AG sponges mimicked the native tissue in terms of homogeneity, balance between the two components, interactions between components, and protein secondary structure. In particular, in the Fourier transform infrared spectrum of the AG scaffold, some of the typical absorption peaks of alginate and gelatin were found to shift toward slightly higher frequencies compared with pure components, suggesting the formation of hydrogen bonds between gelatin and alginate. These interactions can play an important role in cellular response, given that molecular interactions between proteins and polysaccharides in native tissues strongly influence the normal physiology of animals and humans [1]. In addition, the correlation maps, between the chemical maps and the medium spectrum acquired by chemical imaging, showed correlation values close to 1, indicating a high chemical homogeneity of the sponges.
During the swelling test, the developed AG scaffolds showed a high water sorption capacity, indicating a good hydrophilicity degree (swelling at a plateau of about 70%), similar to that of the ECM, which is essential for adequate cell colonization.
Mechanical characterization was carried out on sponges using a dynamic mechanical analyzer (DMA) (Table 1) and compared with values obtained on samples of decellularized porcine myocardium [1]. The sponges resulted stiffer than the native myocardium, showing higher storage and loss modulus, and this may offer several advantages, including reduction of infarct expansion, attenuation of left ventricle remodeling, and amelioration of global left ventricle function [22, 23]. Therefore, AG sponges could improve the outcome of a myocardial tissue engineering procedure, by decreasing heart wall stress [24].
1 Hz | 3.5 Hz | 10 Hz | ||||
---|---|---|---|---|---|---|
E’(x 104 Pa) | E”(x 104 Pa) | E’(x 104 Pa) | E”(x 104 Pa) | E’(x 104 Pa) | E”(x 104 Pa) | |
AG | 70.1 ± 0.2 | 2.1 ± 0.1 | 72.2 ± 0.2 | 2.7 ± 0.2 | 72.9 ± 0.4 | 3.2 ± 0.2 |
AGE | 25.1 ± 0.6 | 1.6 ± 0.1 | 26.1 ± 0.2 | 2.6 ± 0.2 | 27.6 ± 0.4 | 6.6 ± 0.5 |
PDO-reinforced AG | 767 ± 1 | 28 ± 1 | 790 ± 1 | 31 ± 2 | 799 ± 1 | 34 ± 3 |
In vitro proliferation and differentiation tests were performed using C2C12 skeletal myoblasts, as a model of a possible cell source for myocardial regeneration. It was observed that 7 days after seeding the level of cell proliferation on AG sponges was comparable to that of cells directly cultured in the wells of the tissue culture plates (TCPs, positive control) [1]. Cell differentiation was also evaluated, by measuring both actin filament length and the number of nuclei per cell [21, 25]. Five days after seeding, both parameters were significantly higher on the AG sponges than on positive control, suggesting the ability of the AG scaffolds to promote and support myoblast differentiation.
In addition, a polydimethylsiloxane microfluidic bioreactor was designed for perfusion culture of cardiomyocytes seeded onto the AG sponges, to evaluate the cellular responses under dynamic conditions. Cell viability was evaluated for up to 14 days post seeding. The majority of the seeded cardiomyocytes were able to stay alive under the dynamic culture, thanks to significantly better nutrient transport provided by the perfusion. Interestingly, on some samples, spontaneous beating was observed by video microscopy at later stages of culture.
Overall, the results obtained showed a good similarity of the AG sponges with the native tissue in terms of chemical composition, porous morphology, and hydrophilicity degree [1]. This similarity, according to our hypothesis, is based on the strong molecular interactions occurring between gelatin and alginate. In particular, as demonstrated by the infrared analysis, these interactions could produce in gelatin a conformation similar to that of non-fibrillar collagens, which are known to play a key role during fetal cardiac tissue development [26, 27].
As a further step towards the development of a biomimetic scaffold material, a three-component system, combining alginate, gelatin and elastin, was also investigated [7]. Alginate/gelatin/elastin (AGE) sponges (with a 10/80/10 weight ratio among components) were produced by freeze-drying and cross-linked by exposure to glutaraldehyde vapors and subsequent treatment with calcium chloride solution. After cross-linking treatments, samples were immersed in a coagulation bath, to promote ionic interactions among the components.
SEM micrographs of AGE sponges showed a high and homogeneous porosity, with adequate pore interconnectivity (Fig. 1b) [7].
Infrared analysis of the produced sponges showed the displacement of the typical absorption peak of elastin toward a higher frequency, as well as the displacement of the typical band of alginate [7]. These results suggested the presence of interactions (hydrogen bond type) between the protein and the polysaccharide components [28], which were already observed for AG scaffolds [1]. The correlation map between the chemical map and the medium spectrum showed a correlation value around 1 on all the analyzed samples, demonstrating a good chemical homogeneity [7]. The advantage offered by the homogeneous distribution of scaffold components is to avoid the presence of regions with different characteristics, therefore promoting a homogenous effect on cell response. Moreover, the distribution of ECM components is similarly homogeneous in native cardiac tissue, as reported in the literature [1].
The existence of strong interactions between the two proteins was further confirmed by thermal analysis, that pointed out a single glass transition event at a temperature intermediate between those of gelatin and elastin [7].
The mechanical characterization of AGE sponges was performed by DMA and results are collected in (Table 1). Both values of storage modulus (E’) and loss modulus (E”), were higher for AGE sponges [7] than for native myocardium [1]. However, a significant decrease in both E’ and E” with respect to AG sponges was produced by the addition of elastin, which is less stiff than alginate and gelatin [29]. Therefore, even if AGE scaffolds were still stiffer than the native myocardium, their viscoelastic properties resulted closer to that of the native tissue with respect to sponges based on two components only (alginate and gelatin).
Overall, obtained results demonstrated adequate morphological, physicochemical, functional and biological properties for cross-linked AG and AGE sponges [1, 7]. However, although scaffolds based on natural polymer blends represent a promising option for cardiac tissue engineering, if not appropriately reinforced, they lack adequate mechanical strength to withstand the surgical procedures. In particular, preliminary suturability tests, carried out on AG sponges following a procedure described in literature, pointed out inadequate resistance to suture. In order to overcome this limitation, we developed two different strategies for AG sponges reinforcement. In the first strategy, a fibrous poly(caprolactone) (PCL) mesh, produced by dry spinning, was introduced in the center of the sponge (Fig. 2a) [30]. Alternatively, sponges were reinforced by the inclusion of a thin and porous poly(dioxanone) (PDO) membrane in the core of the sponge (Fig. 2b) [9]. Both strategies resulted efficacious in improving mechanical properties (Table 1) and suturability characteristics, without compromising the good properties of the sponges in terms of biocompatibility.
3. BIOMIMETIC SCAFFOLDS BY SURFACE FUNCTIONALIZATION STRATEGIES
3.1. Covalent Modification
Surface modification through the immobilization of short peptide sequences, recognized by cell surface receptors known as integrins, is one of the most investigated strategies to improve the interactions between polymeric scaffolds and cells. These peptides should be stably linked to the surface of the material by means of covalent bonds exploiting the presence of functional groups like hydroxyl-, amino-, or carboxyl groups. The most common strategy to bind peptides to polymer surfaces involves the reaction between substrate carboxylic groups and amino groups of the bioactive molecule, to generate a covalent amide bond.
Membranes for applications in cardiac tissue engineering were produced by casting starting from solutions of PCL and poly(caprolactone)-poly(ethylene oxide)-poly(caprolactone) (PCL-POE-PCL), a block copolymer synthesized in our laboratories [4]. These membranes were functionalized by means of alkaline hydrolysis and subsequent coupling of bioactive molecules through 1-(3-dimethylaminopropyl)-3-ethylcarbodimide hydrochloride/N-hydroxysuccinimide (EDC/NHS) chemistry.
Two pentapeptides, H-Gly-Arg-Gly-Asp-Ser-OH (GRGDS) from fibronectin and H-Tyr-Ile-Gly-Ser-Arg-OH (YIGSR) from laminin, were used as bioactive molecules for the functionalization of the membranes. The GRGDS peptide is known to promote the adhesion of several cell types, including myoblasts, and to stimulate integrins relevant to early cardiac development (α5β1, αvβ3) [31, 32]. YIGSR has also been studied extensively as an adhesion peptide [33, 34]. In addition, laminin has been demonstrated to increase the ability of stem cells to differentiate into beating cardiomyocytes [35], and therefore the YIGSR laminin sequence could be involved not only in adhesion but also in differentiation processes.
For surface functionalization of polymeric membranes, a procedure based on 4 steps was developed: (1) alkaline hydrolysis for the formation of COO- groups on the polymeric surfaces; (2) protonation with HCl; (3) activation of carboxyl groups using the coupling reagents EDC/NHS; and (4) coupling of the peptide sequences. The functionalization procedure was optimized in terms of hydrolysis time, temperature, and sodium hydroxide concentration, for the different substrates [4].
Then, the functionalized materials underwent a complete morphological and physicochemical characterization, to assess the occurrence of the coupling reaction and to study the effect of the functionalization procedure on materials properties. To verify the ability of the functionalized materials to improve cell-material interactions, preliminary in vitro cell culture tests were also performed.
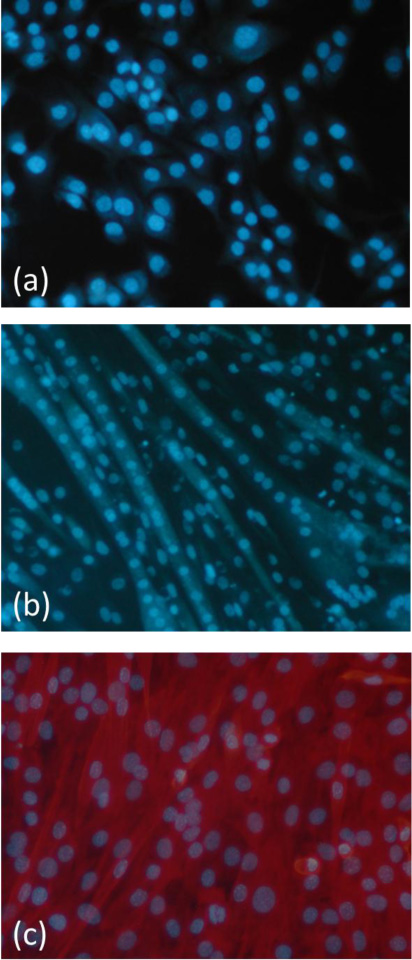
The successful attachment of GRGDS and YIGSR peptides to material surfaces was verified by infrared analysis, which also demonstrated the homogeneous distribution of the biomolecules on the functionalized surfaces [4]. On the basis of the reference values reported in the literature [36], the amount of immobilized peptide (on the order of 10−7 mol/cm2.) resulted to be sufficient to affect cell adhesion and proliferation.
Functionalized substrates were able to promote C2C12 myoblasts adhesion and proliferation, better than untreated materials [4]. The increase in proliferation was particularly evident starting from the third day after seeding, when the number of cells on functionalized materials exhibited at least a twofold increase with respect to the untreated samples and became even higher than the TCPs control. In particular, the GRGDS sequence showed higher efficiency than YIGSR in promoting C2C12 myoblasts growth. On the other side, starting from 7 days after seeding, the appearance of multinucleated myotubes was observed on polymers functionalized with YIGSR, in the absence of the differentiation medium, suggesting a possible action of this laminin sequence in promoting myoblasts differentiation (Fig. 3a and b).
3.2. Modification by Molecular Imprinting
3.2.1. Biodegradable Synthetic Polymers Functionalized by MIP Deposition
A totally new approach for the development of bioactive scaffolds is represented by the application in tissue engineering of Molecular Imprinting (MI) nanotechnology.
MI [37-39] allows the introduction, into a polymeric material, of recognition sites for specific molecular species (templates). Recognition nanocavities can be created through two different strategies: i) the copolymerization of a monomer with a cross-linking agent in the presence of a template [40, 41] or ii) the dissolution of a preformed polymer in a solution containing the molecule to be recognized [42]. In both cases, the spatial arrangement is maintained by the imprinted polymer after template extraction, thanks to crosslinking and consequent matrix rigidity, and confers a selective memory toward the template molecule.
Our research group proposed for the first time MI as a new strategy for the creation of bioactive support structures for cell adhesion and proliferation [5, 6]. Because MI cannot be used directly on biodegradable polymers, essential for tissue engineering, our idea was to use biostable MIP in the modification of degradable scaffolds that would retain their ability to act as temporary supports for cell growth. In order to promote cell-material interactions, particles with molecularly imprinted nanocavities capable of recognizing and rebinding peptide sequences of the ECM proteins (e.g., fibronectin, laminin) secreted by cells or contained in the culture medium were synthetized. After deposition on the scaffold, when the imprinted particles will be exposed to the biological environment or cell culture environment, they will produce an enrichment of the desired ECM protein on the scaffold (Fig. 4 a). Consequently, cell receptors could interact with the rebound protein, promoting a desired cellular response.
The fibronectin pentapeptide GRGDS [5] and the laminin sequence YIGSR [6] were used as template molecules. Fibronectin is the main ECM adhesive glycoprotein which plays a fundamental role in cell adhesion, migration, and repair processes [43], while laminin is a glycoprotein of the basal lamina, able to support and stimulate migration, adhesion, growth, and differentiation of various cell types [44-47].
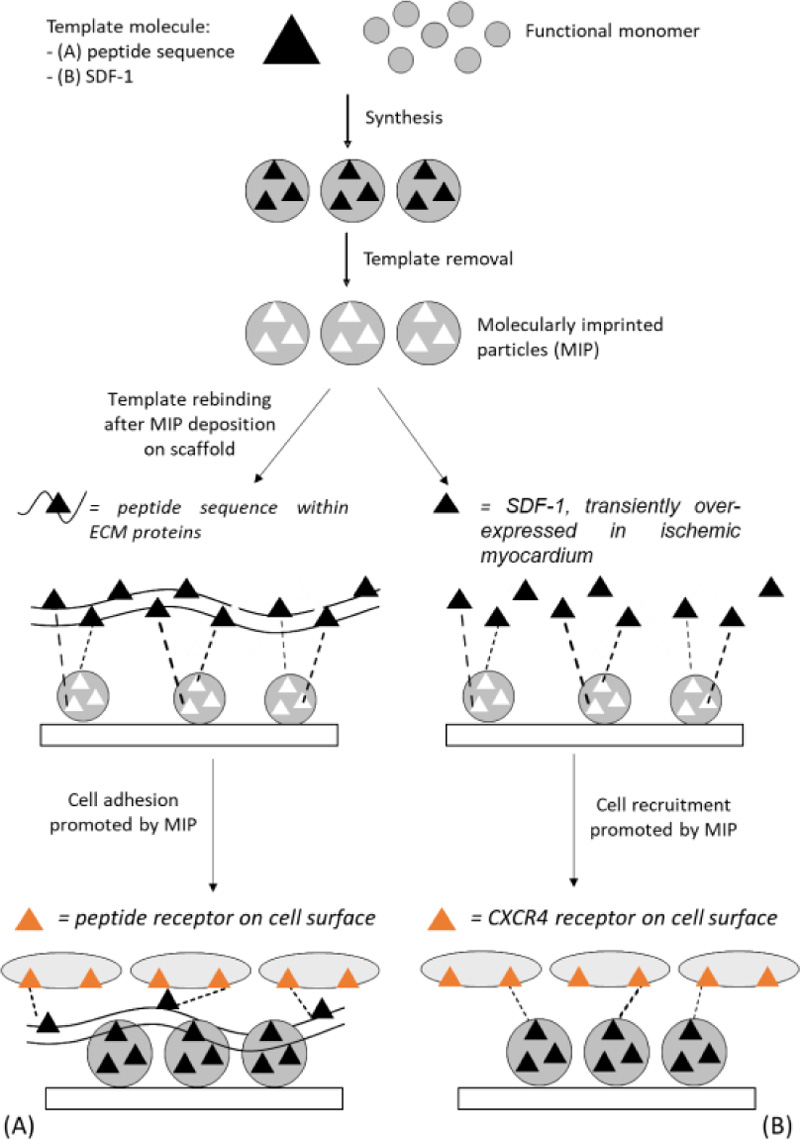
MIP were prepared by a precipitation polymerization method starting from a dilute solution of methacrylic acid (MAA) as the functional monomer in the presence of the template molecule, GRGDS or YIGSR, and an appropriate crosslinking agent, pentaerythritoltriacrylate (PETRA) for GRGDS [5] and trimethylolpropane trimethacrylate (TRIM) for YIGSR [6], to provide sufficient rigidity to the recognition sites in the polymer structure. After MIP synthesis, the template molecule was removed from particles by solvent extraction. Non-imprinted (control) particles (CP) were prepared with the same procedure but in the absence of the template molecule.
The results of MIP characterization showed that the amount of the template molecule contained in the final product, calculated as the difference between the amount contained in the polymerization solution before and after polymerization, was very high (86.1% for GRGDS [5] and 80.8% for YIGSR [6]). The entrapped template was quantified as 22.4 µmol of GRGDS/g of particles [5] and 57.3 µmol of YIGSR/g of particles [6].
The template molecules were removed from the particles by washing them in a Soxhlet apparatus with a methanol/acetic acid mixture. The amount of template removed from the particles was not complete (61% for GRGDS [5] and 54% for YIGSR [6]) as a consequence of several factors, including the high crosslinking degree, the rigidity of the matrix and the establishment of interactions (hydrogen bonds) among the peptides and the functional monomer, MAA.
SEM micrographs showed that, in the case of GRGDS, MIP and CP were composed of densely fused microgel particles having the same shape and size (1µm diameter) [5]. In the case of YIGSR, a powder of nanosized particles was observed for CP, while MIP appeared as aggregates of nanoparticles, with an average diameter of about 200 nm [6].
Releasing tests were performed by maintaining MIP in contact with the physiological solution and the released template was quantified by HPLC analysis of the releasing solution. Obtained results showed that no peptide was released after extraction, confirming that the residual template (after the removal process) remains entrapped into the matrix [6]. This turns out to be very beneficial for tissue engineering applications, since the presence of free peptides in solution, instead of linked to a surface, could inhibit cell proliferation, as reported in the literature [44].
The performance of the obtained particles in rebinding the specific template molecule was investigated by recognition tests. Briefly, MIP and CP were put in contact with a template solution (the so-called rebinding solution), under stirring, for three cycles of 30 minutes each. At the end of each cycle, the residual peptide concentration in the rebinding solution was determined by HPLC analysis. Both MIP systems were found to be more efficient in template recognition than the related controls, confirming the formation of specific binding sites in the polymer network [5, 6]. Moreover, the amounts of rebound templates were comparable with those reported for more rigid and smaller templates, such as theophylline [48].
A cytotoxicity test was performed by culturing C2C12 mouse skeletal myoblasts in a culture medium conditioned with MIP extracts [5, 6]. The results of in vitro cytotoxicity tests on MIP extracts showed no cytotoxic activity against C2C12 myoblasts.
Thanks to the positive outcome of MIP characterization, they were used for the functionalization of polymeric films [5, 6]. In particular, MIPs were deposited on the surface of PCL, PCL-POE-PCL, and poly(ester urethane) (PU) biodegradable films prepared by casting [5, 6]. The amount of MIP deposited on polymeric films was chosen to have a superficial density of 1 nmol of peptide/cm2, which could be considered sufficient to promote cell-material interactions, as reported in the literature [36]. Polymeric films on which CP were deposited were used as controls.
SEM micrographs of MIP-modified films showed a quite homogeneous distribution of the particles on the substrates [5, 6].
Recognition tests performed on functionalized films showed that the deposition of the imprinted particles did not alter their specific recognition and rebinding behavior [5, 6]. Moreover, the functionalized films showed a higher quantitative binding than MIP alone, suggesting the creation of a preferred microenvironment for the rebinding process.
In vitro cell culture tests with C2C12 myoblasts were carried out to evaluate the biocompatibility of MIP-modified polymeric films and to verify the ability of this new functionalization method to improve cell adhesion and growth. MIP-modified polymeric films significantly increased cell proliferation with respect to non-functionalized materials, rising values very close to that offered by the positive control (TCPs), starting from the second day after seeding [5, 6]. The reason for this result could be found in the type of cell-material interactions promoted by MI. In contrast with traditional functionalization strategies, in which bioactive molecules are exposed to scaffold surface and, therefore, cell adhesion is directly promoted, when MIP are used as functionalization devices, the cell-material interactions are indirectly promoted, once the ECM proteins contained in the culture medium or secreted by the cells are recognized and rebound by molecularly imprinted nanocavities. In particular, MIP with recognition properties towards YIGSR appeared capable of promoting myoblast differentiation, as demonstrated by the appearance of multinucleated myotubes during the proliferation test, in the absence of the differentiation medium (Fig. 3c) [6].
The results of these studies indicate that MI is a promising strategy for scaffold functionalization and it could be useful for creating systems capable of promoting the tissue regeneration process [5, 6].
3.2.2. Biomimetic Scaffolds based on Natural Polymers, Functionalized by MIP Deposition
In a recently published paper, biomimetic scaffolds were designed and manufactured by combining two different strategies: i) the use of a protein/polysaccharide mixture, mimicking the composition of native ECM, as scaffold material (as already described in paragraph 2) and ii) scaffold functionalization by MI [7].
MIP with recognition properties towards two different peptides, H-Gly-Arg-Gly-Asp-Ser-Pro-OH (GRGDSP) from fibronectin and YIGSR from laminin were synthesized by precipitation polymerization of MAA, using PETRA as a cross-linking agent [7]. The two MIP systems will be indicated hereafter as MIP-GRGDSP and MIP-YIGSR, respectively. CP were also prepared under the same conditions but in the absence of the template molecule. The GRGDSP sequence was chosen as a template because, as the GRGDS sequence previously described, it is able to promote cell adhesion; moreover, it can stimulate integrins involved in early cardiac development. For what concerns MIP with recognition properties towards the laminin sequence YIGSR, here PETRA was used as a cross-linker instead of TRIM [6] during particle synthesis, with the aim to improve the rebinding performance, as template affinity is greatly influenced by the nature of the cross-linker. AGE sponges, described in section 2, were then functionalized by deposition of MIP on their surface. A complete morphological, physicochemical, mechanical, functional, and biological characterization was performed on functionalized sponges [7].
MIP with a spherical shape and an average diameter of 1 µm were obtained for both formulations [7]. High values of monomer conversion and template entrapment were observed [7]. The template molecule was only partially removed from the particles (percentage of the extracted template below 50%), as typically occurs with a protein template. The infrared analysis confirmed the desired chemical structure of MIP, as well as successful template rebinding. Good recognition and rebinding capabilities were demonstrated, as both the recognition factor and the selectivity factor resulted much higher than 1 [7]. With specific reference to MIP-YIGSR, comparing the results obtained in [7] with those previously reported [6], the quantitative binding was increased, while the percentage of unspecific adsorption with respect to total adsorption was significantly decreased (from 83.3% in [6] to 60.0% in [7]). As a consequence, an increase in the recognition factor (1.66 in [7] against 1.2 in [6]) was reported. These results could be explained by the different cross-linker used. As already reported in the literature [49], template rebinding is influenced by the cross-linker used during MIP synthesis, and in particular, recognition properties are improved when PETRA is used instead of TRIM.
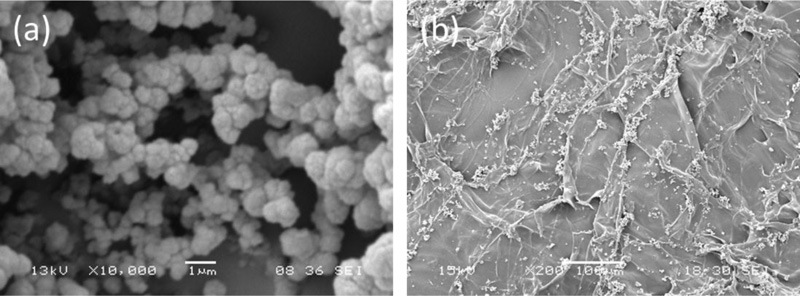
Therefore, MIP-YIGSR and MIP-GRGDSP were used for the functionalization of AGE scaffolds [7].
The morphological analysis showed a quite homogeneous distribution of MIP aggregates on scaffold surface (Fig. 5), without significant difference between the two different types of particles [7].
The deposition of MIP-GRGDSP and MIP-YIGSR on polymeric sponges did not alter their specific recognition and binding behavior [7]. Comparing these results with those obtained for not deposited MIP, sponges functionalized with MIP showed a higher quantitative binding than free particles, suggesting the creation on the sponges of a preferred microenvironment for the rebinding process. A higher quantitative binding was found for scaffolds functionalized with MIP-YIGSR with respect to those functionalized with MIP-GRGDSP, attributable to the higher percentage of template extraction, which increases the number of imprinted cavities available to rebind the template.
The in vitro biological characterization was carried out by using rat cardiac progenitor cells (rCPCs). The in vitro quantitative evaluation of the fractional area occupied by cells documented the presence after 72 h of rCPCs on AGE sponges, in both functionalized and not functionalized forms [7].
In order to test the in vivo properties of functionalized sponges, experiments were performed on a cryoinjury rat model [7]. In vivo study revealed that progenitor cells were still present within AGE sponges 10 days after their implant. Cell differentiation toward myocardial adult phenotypes was immunohistochemically detectable. In particular, it was observed that MIP-GRGDSP and MIP-YIGSR functionalization fostered the expression of α-SARC and vWF proteins, suggesting an important role of implanted AGE sponges in the differentiation of seeded rCPCs toward myocytes and endothelial cells, respectively. The detection of β1-integrin on cells located within the scaffolds supported the hypothesis concerning the ability of the sponge to actively interact with the native myocardium and promote cell adhesion.
This study was particularly significant, as it demonstrated that the combination of a biomimetic substrate with imprinted particles capable of imparting recognition properties to the scaffold, represents an innovative and promising approach in the field of cardiac tissue engineering that allows to obtain a functionalized system able to promote the desired cellular responses.
4. BIOMIMETIC SCAFFOLDS BY LOADING AND RELEASING OF BIOACTIVE AGENTS
4.1. Growth Factor Loading in Three-Dimensional Preformed Scaffolds by Avidin-Biotin Binding Strategy
In the development of bioactive scaffolds for tissue engineering applications, one of the most investigated approaches is the “decoration” of the scaffold with GFs. However, a problem, still not perfectly solved, is that concerning the loading of these factors, which are easily susceptible to denaturation or inactivation. The avidin-biotin binding system could represent a valid strategy, which avoids the use of high temperature or harsh solvents for GFs immobilization. Avidin is a tetrameric glycoprotein found in egg white that binds strongly with biotin molecules. The avidin-biotin interaction is used to immobilize biomolecules on different surfaces, for several applications [50] including tissue engineering [51, 52].
Therefore, the avidin-biotin binding strategy was investigated by us to immobilize Insulin-like growth factor-1 (IGF-1) on the surface of AG sponges, reinforced with a PDO membrane to improve their suturability [9]. Using a biotinylated gelatin during scaffold preparation, we produced substrates exposing biotin molecules on their surface. Avidin was then used as a bridge between biotin on scaffold surface and a biotinylated IGF-1. This growth factor was chosen because of its ability to promote survival, homing, engraftment, and differentiation of cardiac progenitor cells as well as to improve revascularization [28, 53, 54].
The successful binding of IGF-1 on scaffold surface was verified by infrared analysis [9]. After growth factor binding, the characteristic absorption peak of avidin decreased, because of the presence of the bioactive molecule.
In vitro biological tests showed that IGF-1-functionalized scaffolds increased cell adhesion, promoted intercellular communication and favored full colonization of scaffolds by rCPCs [9].
In vivo tests showed that scaffold decoration with biotinylated IGF-1 promoted cell retention and reduced LV dilatation (Fig. 6a) [9].
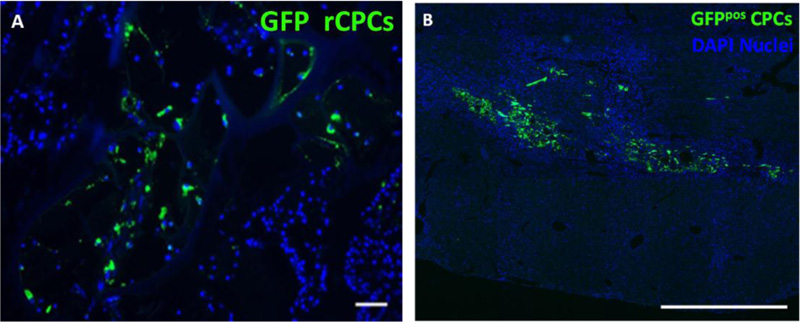
All these findings suggest that the avidin-biotin binding system, used for the first time by us for the modification of three-dimensional preformed scaffolds, is an effective strategy for their functionalization with bioactive molecules. Combining this strategy with the use of different bioactive molecules, it could be possible to create a complex microenvironment, controlling the endogenous regeneration of the injured myocardium.
4.2. Growth Factor Loading in Injectable Scaffolds
An alternative to the traditional in vitro cardiac tissue engineering strategy is the in vivo approach, based on the implantation of an unseeded scaffold or injectable biomaterial on the damaged myocardium, to create a favorable environment for cell recruitment, proliferation and differentiation, thus allowing the regeneration of the injured tissue in its natural milieu [55]. A key step for a successful in vivo approach is scaffold functionalization, as the release of bioactive molecules (such as GFs) to the surrounding cells [56] could promote endogenous tissue regeneration.
Injectable scaffolds in form of microparticles can offer several advantages over other injectable systems, including a high surface to volume ratio and the possibility of cell seeding before transplantation. Therefore, we produced in our laboratories a microspheres-based bioactive injectable scaffold for in vivo cardiac tissue engineering [8].
Microspheres based on a gelatin/gellan (Gel/GE) blend were obtained by a water-in-oil emulsion, using phosphatidylcholine, a phospholipid from biological membranes, as surfactant [8]. The two natural polymers were chosen because of their marked capability to absorb water [57], which makes them very similar to soft tissues, as the myocardium. Moreover, their hydrogel nature can favor the controlled release of bioactive agents [58]. Gel/GE microspheres were functionalized by loading with IGF-1 [8]. The IGF-1 loading procedure was optimized to obtain a sustained release from the microspheres over a few days. To avoid denaturation that could occur during the emulsion process, IGF-1 was loaded by adsorption on preformed microspheres during the cross-linking process [8].
All microspheres presented a spherical shape and a porous surface [8]. No significant morphological variation was observed for IGF-1 loaded particles. An average diameter of 66+17 µm was measured for dried particles while the average wet diameter was equal to 123+24 µm. These dimensions are suitable for injection through a narrow needle; moreover, being the swollen diameter below 200 µm, this should enhance nutrient and waste exchange, maintaining high cell viability [59].
The results of Chemical Imaging analysis performed after microspheres loading with IGF-1 showed that the growth factor was distributed not only on microsphere surface, but also within the entire microsphere volume [8].
IGF-1 releasing tests showed a burst release during the first 6 hours, attributable to IGF-1 loaded on the surface, followed by a slower release, completed in 6 days, due to the diffusion of IGF-1 distributed within microspheres matrix [8].
In vitro cell culture tests showed that IGF-1 funtionalized microspheres promoted rCPCs adhesion, and cluster formation [8]. After dynamic suspension culture within an impeller-free bioreactor, cells still adhered to microspheres, spreading their cytoplasm over microsphere surface.
Intramyocardial administration of microspheres in a cryoinjury rat model attenuated chamber dilatation, myocardial damage and fibrosis and improved cell homing (Fig. 6b).
4.3. Promoting Scaffold Enrichment with Bioactive Molecules by MI
In vivo cardiac tissue engineering aims to regenerate the infarcted myocardium by recruitment of endogenous cells and subsequent stimulation of the regeneration process. The development of a bioactive scaffold, able to stimulate cell recruitment and consequently tissue repair at the site of damage, is therefore of paramount importance. In particular, myocardial regeneration can be stimulated by the delivery of cytokines that induce the recruitment of stem cells which, upon engraftment and differentiation toward myocardial phenotypes, lead to tissue growth. Among these cytokines, the stromal-derived factor-1 (SDF-1) is the most potent chemoattractant agent for stem cells that possess the specific receptor CXCR4, including cardiac progenitor cells (CPCs) [60]. SDF-1 also stimulates cell retention, growth and proliferation [61-63]. In addition, it has been reported to decrease apoptosis and increase cell survival in the infarct zone [64, 65], as well as to improve heart function and survival after myocardial infarction [64-66].
Traditionally, GFs are physically loaded into the scaffolds, directly in the scaffold matrix or through the use of carriers, so that they can be released from the scaffold and trigger or modulate new tissue formation.
Recently, we have proposed MI nanotechnology as an alternative and innovative functionalization strategy in the development of bioactive scaffolds. MIP with recognition properties towards SDF-1 (hereafter indicated as MIP-SDF-1) were synthesized, characterized and used for the functionalization of biomimetic AGE sponges [10]. The idea is that the combination of biomimetic AGE sponges with molecularly imprinted particles could have a synergistic effect on cardiac repair: in addition to providing a native-like environment, functionalization by deposition of MIP-SDF1 is expected to allow an enrichment of the scaffold of SDF-1, to favour the migration and retention of stem cells at the site of injury and promote myocardium healing (Fig. 4b).
AGE sponges were fabricated by freeze-drying and subsequently cross-linked by calcium ions and glutaraldehyde, as previously described. MIP-SDF1 were obtained by precipitation polymerization, using the SDF-1 molecule as a template, in the presence of a cross-linker (TRIM). Template extraction was performed by repeated washings with PBS, under vigorous stirring. Sponges were functionalized by MIP-SDF1 deposition on their surface. Morphological, physicochemical and functional characterization was carried out. In addition, a preliminary in vitro biological investigation was performed to verify the MIP-SDF1 effect on rCPCs.
The obtained MIP-SDF1 particles were well separated, with a spherical shape and a diameter ranging between 0.6 and 0.9 µm [10]. Template removal from the particles was not complete (73%), probably as a consequence of the high cross-linking degree and the hydrogen bond interactions occurring between the monomer and the template [10]. Recognition tests showed that MIP-SDF1 were able to recognize and rebind the template, with a recognition factor of 1.59 [10]. MIP were also able to distinguish between the template and an analogue molecule, with a selectivity factor (2.4) and a specific selectivity factor (2.22) both higher than 1.
Infrared Chemical Imaging analysis of the functionalized sponges pointed out a sufficiently homogeneous MIP-SDF1 distribution on scaffold surface [10].
Recognition tests performed on MIP-modified scaffolds showed that MIP maintained their specific recognition and binding behaviour after deposition on polymeric sponges [10]. However, values of quantitative binding were lower than those obtained for free nanoparticles. This could be explained by the fact that when imprinted nanoparticles are deposited on the sponges, not all the recognition nanocavities remain easily accessible for the rebinding process, considering also the large dimension of the protein template. Nevertheless, the amount of rebound SDF-1 can be considered sufficient to promote a desired cellular response.
Results of in vitro CPC culture tests were encouraging, showing that cell adherence and retention were promoted by MIP-SDF1 functionalization [10].
5. Biomimetic Scaffolds Mimicking Cardiac ECM Microarchitecture
Among the different scaffold properties influencing cell behavior, there are also microarchitecture and surface topography [67], as in native tissues the organization of cells and ECM is strictly related to tissue function.
Therefore, over the last years, many microfabrication techniques, such as soft lithography, selective laser sintering, and fused deposition modelling [67-74], have been developed in order to reproduce in the scaffold the typical cell and ECM spatial organization of natural tissues.
Our idea was to use the soft lithography technique to produce 3D microfabricated scaffolds, with cardiac ECM-like architecture [11]. First of all, in order to identify the main features of the cardiac ECM structure, we prepared samples of decellularized porcine myocardium and we observed them under an optical microscope. The obtained information was used to draw a simplified model of cardiac ECM, which was then reproduced through a soft lithographic process. Image processing allowed us to create a 3D model of cardiac ECM, composed of rectangular cells with 100×500 μm dimension of line length and 30 or 70 μm of line width (Fig. 7a, b).
Scaffolds were then produced using a bioartificial blend [75], based on alginate and gelatin in combination with a novel thermosensitive and bioresorbable copolymer, synthesized in our laboratories [76]. Topological and mechanical properties of the obtained systems were investigated and preliminary cell studies were performed using the C2C12 myoblasts cell line [11].
SEM micrographs showed that microfabricated scaffolds faithfully reproduced the topology of the cardiac ECM model designed by us (Fig. 7c).
The results of the mechanical characterization showed that the scaffold has anisotropic properties, with different mechanical responses depending on the direction in which the load is applied. The associated anisotropy ratio (ELONG/ ETRANS) was equal to 1.9 [11]. Scaffold anisotropy is a fundamental requirement for myocardial tissue repair. The ventricular myocardium is an anisotropic tissue, with tensile mechanical properties dictated by cardiac muscle fiber orientation [77].
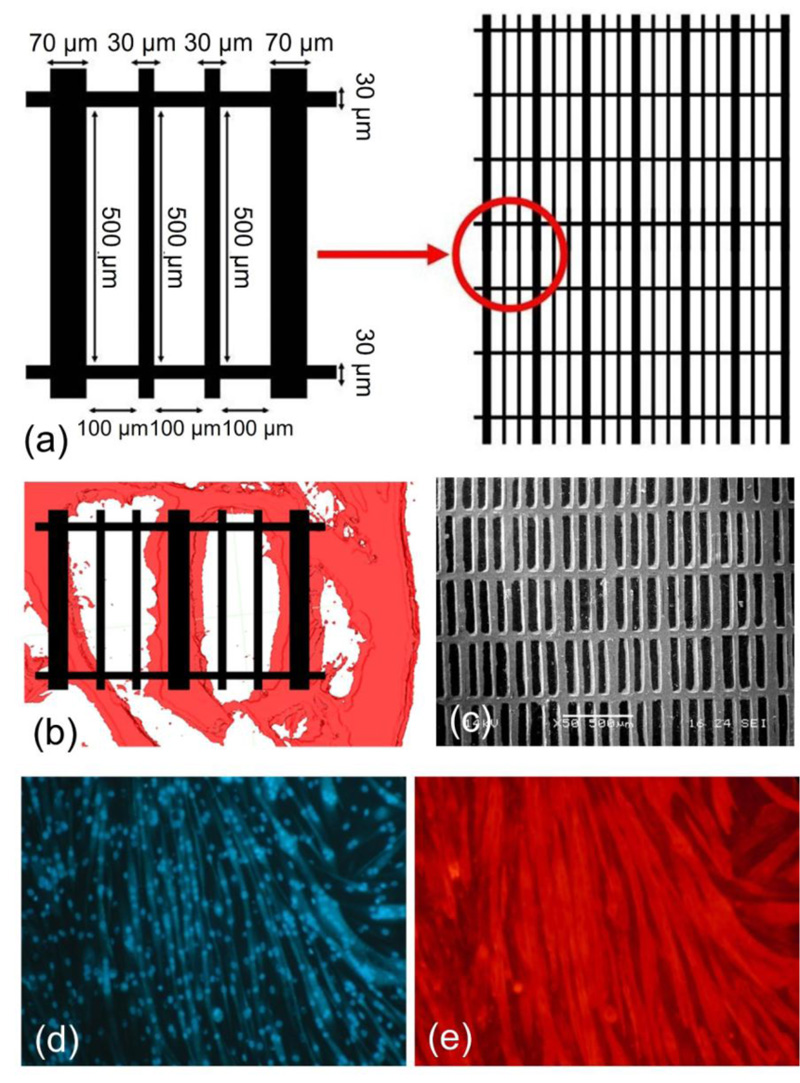
Moreover, comparing the obtained values [11] with those of the human left ventricular myocardium reported in the literature [78, 79], it can be observed that the human myocardium is similarly anisotropic but less stiff. As already underlined, this greater stiffness can however be considered an advantage for the simultaneous application of the construct as a stem cell delivery vehicle and mechanical support to the ventricle. It has been in fact demonstrated in the literature that materials stiffer than the native myocardium are necessary to support the heart and prevent ventricle dilatation after the infarct [79].
With regard to biological tests, it was observed that C2C12 myoblasts adhered to micropatterned scaffolds and cell density increased with the increase of culture time, similarly to control [11]. Seven days after seeding, an improvement in cell proliferation was observed on patterned structures with respect to unpatterned ones, reaching statistical significance and highlighting the cell preference for the patterned structure. The microfabricated scaffolds were also able to support myoblast differentiation, as indicated by the appearance of multinucleated myotubes at day 8 of the differentiation test [11]. As cell culture time increased, there was always a great number of multinucleated myotubes, which aligned along the scaffold lines. These results suggested that the microfabricated scaffolds are able to support not only myoblasts adhesion and proliferation, but also their elongation and alignment (Fig. 7, d-e).
CONCLUSION
Over the past years, the research activity carried out in our laboratories in the field of myocardial tissue engineering, has been mainly based on the fine-tuning and development of scaffolds capable of reproducing as faithfully as possible what is considered as the natural scaffold of the connective tissues: the ECM.
The production of an ECM-like scaffold should take into consideration different aspects, including chemical composition, architecture, and inclusion of bioactive signals.
The first approach was to produce an ECM substitute, composed of natural polymers of different nature, such as alginate, gelatin, and elastin, capable of reproducing the composition of the natural ECM and the interactions that occur within it between the protein and polysaccharide components.
Moreover, several strategies have been investigated to produce bioactive systems, capable of acting as the natural ECM, as a reservoir of GFs, influencing cellular behavior and favoring the formation of new tissue.
In addition, signaling molecules similar to those present in the adhesive proteins of native ECM have been used for the surface functionalization of the scaffolds, using both conventional techniques, such as covalent modification, and innovative methods, such as MI. All the different approaches have produced encouraging results, in terms of ability to promote cellular processes, such as adhesion and proliferation, that are essential for the formation of a new functional tissue.
For what concerns scaffold structure, we produced soft-lithography microfabricated scaffolds mimicking the microarchitecture of native ECM and therefore able to promote a preferential cell organization.
A very significant example, recently published, is related to the design and fabrication of biomimetic scaffolds, by combining the use of a natural polymer blend, reproducing the protein/polysaccharide mixture typically present in the ECM, with the surface functionalization of the system through the deposition of MIP, able to recognize and rebind two different peptide sequences from ECM adhesive glycoproteins, fibronectin and laminin.
From an overall analysis of the research activity carried out, it emerges that the most promising approach for promoting myocardial tissue regeneration, through the use of a scaffold-based tissue engineering paradigm, is the combination of different strategies, all aimed at the production of biomimetic and bioactive support systems, capable of communicating with cells by mimicking as much as possible the composition, microstructure, and functions of the natural ECM.
LIST OF ABBREVIATIONS
AG | = Alginate/Gelatin |
AGE | = Alginate/Gelatin/Elastin |
CPCs | = Cardiac Progenitor Cells |
DMA | = Dynamic Mechanical Analyzer |
ECM | = Extracellular Matrix |
EDC/NHS | = 1-(3-dimethylaminopropyl)-3-ethylcarbodimide hydrochloride/N-hydroxysuccinimide |
Gel/GE | = Gelatin/Gellan |
GFs | = Growth Factors |
GRGDS | = H-Gly-Arg-Gly-Asp-Ser-OH |
GRGDSP | = H-Gly-Arg-Gly-Asp-Ser-Pro-OH |
IGF-1 | = Insulin-like Growth Factor-1 |
MAA | = Methacrylic Acid |
MI | = Molecular Imprinting |
MIP | = Molecularly Imprinted Particles |
PCL | = Poly(caprolactone) |
PCL-POE-PCL | = Poly(caprolactone)–Poly(ethylene oxide)–Poly(caprolactone) |
PDO | = Poly(dioxanone) |
PETRA | = Pentaerythritoltriacrylate |
PU | = Poly(ester urethane) |
rCPCs | = rat Cardiac Progenitor Cells |
SDF-1 | = Stromal-Derived Factor-1 |
TCPs | = Tissue Culture Plates |
TRIM | = Trimethylpropane Trimethacrylate |
YIGSR | = H-Tyr-Ile-Gly-Ser-Arg-OH |
CONSENT FOR PUBLICATION
Not applicable.
AVAILABILITY OF DATA AND MATERIALS
The authors confirm that the data supporting the findings of this research are available within the articles mentioned as references.
FUNDING
None.
CONFLICT OF INTEREST
Dr. Elisabetta Rosellini is Associate Editorial Board Member of The Open Biomedical Engineering Journal.
ACKNOWLEDGEMENTS
Declared none.