All published articles of this journal are available on ScienceDirect.
Physiological Microenvironmental Conditions in Different Scalable Culture Systems for Pluripotent Stem Cell Expansion and Differentiation
Abstract
Human Pluripotent Stem Cells (PSCs) are a valuable cell type that has a wide range of biomedical applications because they can differentiate into many types of adult somatic cell. Numerous studies have examined the clinical applications of PSCs. However, several factors such as bioreactor design, mechanical stress, and the physiological environment have not been optimized. These factors can significantly alter the pluripotency and proliferation properties of the cells, which are important for the mass production of PSCs. Nutritional mass transfer and oxygen transfer must be effectively maintained to obtain a high yield. Various culture systems are currently available for optimum cell propagation by maintaining the physiological conditions necessary for cell cultivation. Each type of culture system using a different configuration with various advantages and disadvantages affecting the mechanical conditions in the bioreactor, such as shear stress. These factors make it difficult to preserve the cellular viability and pluripotency of PSCs. Additional limitations of the culture system for PSCs must also be identified and overcome to maintain the culture conditions and enable large-scale expansion and differentiation of PSCs. This review describes the different physiological conditions in the various culture systems and recent developments in culture technology for PSC expansion and differentiation.
1. INTRODUCTION
Human Pluripotent Stem Cells (PSCs) exhibits decent self-renewal and differentiation capability into various cell types consisted of the human body. They show remarkable promise for clinical applications and novel strategies in regenerative medicine, drug discovery, and in vitro toxicology for clinical and industrial purposes. The target organs of these technologies vary widely from small organs such as retinal tissue [1] to large organs such as the heart [2], pancreas [3], and liver [4].
For all of these applications, a sufficient quality and quantity of PSCs are needed, which can be generated in scalable three dimensional (3D) culture system that supports a larger density of cell culture and higher growth rate with good manufacturing practice-compatible, simple, and easy to automate [5-8]. Although numerous research groups have attempted to develop optimum culture systems for PSCs, the currently available culture systems show some limitations, such as difficulties in scaling up, automation, and standardization. Therefore, the effectiveness of these scalable culture technology for PSC expansion and differentiation must be improved.
Here, we introduce the advantages and remaining disadvantages of various culture systems for PSCs mass production and differentiation. In addition, we describe the important microenvironmental conditions required to optimize PSCs mass production systems development as a consideration when selecting, combining, or modifying various culture systems.
2. PLURIPOTENT STEM CELL TYPES
Generally, PSCs can be derived from embryonic or adult tissue and are classified as Embryonic Stem Cells (ESCs) or induced Pluripotent Stem Cells (iPSCs), respectively.
ESCs were firstly isolated from the inner cell mass of blastocysts by Thomson et al. in 1998 [9]. ESCs have some disadvantages, such as the requirement to extract and manipulate the embryo, which is limited by ethical considerations. In clinical use, because ESCs are derived from embryonic stage cells, it is more difficult to obtain immunocompatible cells for transplantation. This increases the risk of immune rejection in allogeneic transplantation. To overcome this limitation, immunosuppression or immuno-isolation such as encapsulation is necessary when ESCs are administered into a recipient’s body. These techniques have been applied in some clinical trials, such as to encapsulate insulin-producing cells from human ESCs during pancreatic regeneration [10].
iPSCs were firstly generated by Yamanaka et al. in 2006 [11, 12]. Adult somatic cells were reprogrammed into pluripotent cells by overexpressing transcription factors Oct4, Sox2, Klf- 4 and c-Myc via transfection into human fibroblasts [13-14]. This achievement is very promising for both regenerative medicine and industrial-related applications such as drug screening. iPSCs not only show similar characteristics such as remarkable pluripotency to ESCs but also present minimum ethical issues such as those involving embryo destruction for ESCs [5, 15, 16]. Moreover, this technology provides the opportunity to generate iPSCs from patients via tissue biopsy, which may minimize immune rejection by using autologous transplantation. In contrast, this reprogramming technology also has drawbacks. The integration of the transcription factor into cells genome can cause an unwanted effect. For instance, the insertion of proto-oncogene like c-myc increases the risk of tumor formation [17]. Currently, numerous studies were attempted to resolve this problem with non-integrating methods [17-25]. However, most of the results show a lack of reprogramming efficiency when compared to integrating methods.
3. DEVELOPMENT OF PSCs CULTURE
The first method for the large-scale production of animal cells was developed for baby hamster kidney cells by Capstick et al. in 1962 [26, 27]. This technology has been applied to a broad range of biological products, such as monoclonal antibodies, hormones, vaccines, and other pharmaceutical products [28]. In PSC cultivation, the purpose of mass production is to prepare a large number of cells for industrial and clinical use. For example, pancreatic islet transplantation requires at least 6 × 108 beta cells which derived from an approximately 0.6 m2 of culture area; this corresponds to 600 6-well plates [29]. To regenerate 30% of liver tissue, 6 × 1010 liver cells are required, covering 60 m2 of culture area or 60,000 6-well plates [30]. To obtain the high-quality cells for these applications, it is important to sustain the self-renewal properties, maintain the pluripotency, and provide cryopreservation to maintain established cultures by using proper microenvironment for cell growth [31].
Selection of the culture system for PSCs largely depends on their cellular characteristic during cultivation (Table 1). In all culture methods, a single hPSC is known to undergo apoptosis without Rho-associated protein kinase (ROCK) inhibition. These conditions are thought to be caused by anoikis, the apoptosis mechanism induced by a lack or alteration of cell-cell or cell-matrix interactions [32]. When hPSCs dissociated into single cells, the loss of E-cadherin, a key molecule in intercellular adhesion, can activate the ROCK-dependent signaling cascade to induce myosin hyperactivation. These cascades can increase myosin contraction, impacting cellular vulnerability and causing apoptosis [33-35]. Thus, hPSCs are typically seeded as clusters or single cells with ROCK inhibitors such as Y-27632 and HA-1077 [36, 37]. Adherent culture is the most widely used method for culturing undifferentiated PSCs. However, they can also be cultured in suspension as single cells or aggregates [38].
The extracellular signaling is also important to maintain the state of pluripotency. Several study revealed several signaling molecule such as Transforming Growth Factor beta 1 (TGF-β1) [39, 40], activin A [39, 41, 42], bone morphogenetic protein 4 (BMP-4) [43, 44], Fibroblast Growth Factor 2 (FGF2) [45], Leukemia Inhibitory Factor (LIF) [43], and Nodal [39, 46]. In order to provide the required amount to keep their self-renewal, these molecules were regularly supplemented in the culture medium.
PSCs Type | Possible Combined Culture Technique | |
---|---|---|
Adherent | Static Culture Systems | Automation |
Hollow fiber bioreactor | Scaffold Perfusion Automation |
|
Stirred culture systems | Microcarrier Cell encapsulation Perfusion Automation |
|
Rotary culture systems | Microcarrier Cell encapsulation Perfusion Automation |
|
Suspension | Stirred culture systems | Aggregates culture Cell encapsulation Perfusion Automation |
Rotary culture systems | Aggregates culture Cell encapsulation Perfusion Automation |
3.1. Adherent Culture Methods
Adherent culture is the most widely used cell culture method for biological studies. For adherent PSCs, the interactions between PSCs and feeder cells are essentially required to provide support, such as producing secreted growth factor, expressing specific ligands, releasing cytokine, and providing the cell-cell interaction for stable attachment which is important for the cell growth and pluripotency [47, 48]. Several proteins which required for maintaining the pluripotency, such as Transforming Growth Factor beta 1 (TGF-β1) [49], activin A [49], bone morphogenetic protein (BMP)-4 [49], Fibroblast growth factor 2 (FGF2) [49], and Wnt-3 [50] were secreted by the feeder cells.
Inactivated mouse embryonic fibroblasts are traditionally used as a supportive feeder layer to sustain hPSC propagation [15, 51, 52]. However, co-culturing PSCs with animal feeder cells present the transmission of unwanted genes, viral contaminations, or immunogenic nonhuman saccharides such as sialic acid [53], as well as variability in experimental results [54]. To overcome these limitations, many studies have developed xeno-free human feeder cells, such as human foreskin fibroblasts [49, 55, 56] human adipose-derived stromal cells [57, 58], amniotic epithelial cells [59, 60], fetal skin cells [61, 62], and amniotic Mesenchymal Stem Cells (MSCs) [63]. Autologous adult human fibroblasts are preferably used as feeder cells in clinical applications because they minimize xenogeneic substances from animals and are easy to obtain. Moreover, human PSCs can be generated from isogenic parental cells as feeder cells, and thus the compatibility of cell-cell interactions can be better maintained [64]. However, maintenance in the culture systems using feeder cells is costly and time-consuming.
Therefore, in large-scale culture, the application of feeder layer can be eliminated to obtain a higher yield and reduce production costs [65]. Alternatively, feeder cells can be replaced with a conditioned medium and Extracellular Matrix (ECM), such as Matrigel [66], fibronectin [66], laminin [67-69], and vitronectin [70], which are normally produced by feeder cells to maintain the PSCs in culture. The medium consists of non-xenogeneic compounds that are also essentially required for the propagation and differentiation of hPSCs used in clinical applications. In addition, the growth factor such as such as activin A, TGF-β1, FGF2, Insulin, Transferrin, and Nodal also added in the medium formulation to support the proliferation and reconstitute its production from the feeder cells [70]. Currently, feeder cell-conditioned media or commercially available synthetic media for feeder-free culture have been used for PSCs culture. Media selection varies depending on the cell type and it is necessary to optimize the media conditions for different culture methods and cells [7, 71, 72].
To harvest PSCs, the conventional adherent method requires cell removal by mechanical or enzymatic separation, which may damage the cells and affect the quality and quantity of mass-produced PSCs [73]. These conditions make the use of human PSCs for clinical applications which were more difficult to achieve.
3.2. Suspension Culture Methods
Current development in cell culture technology has enabled adherent PSCs to be adapted for suspension culture. This method can provide a better mass transfer of nutrition and does not require enzymatic or physical removal, which can disrupt the cell membrane. Elimination of the feeder layer and synthetic matrices can simplify the procedure and minimize contamination. In industrial applications, this system can also increase the scalability by enabling high-density cell culture (> 1 × 107 cells/mL) and significantly reducing the costs involved [28]. A recent experimental study revealed that global gene expression, mainly the pluripotency gene and functional characteristics, were comparable to those maintained under adherence conditions [74, 75]. Differentially expressed genes between suspension and adherent PSCs culture were mainly associated with cellular adhesion and extracellular matrix interactions [74, 76]. In addition, the 3D structure also shows better performance in differentiation in comparison with adherent culture based-differentiation. This phenomenon occurred because of the lack of interaction between PSCs in adherent culture [77].
4. MECHANICAL AND PHYSIOLOGICAL ENVIRONMENT IN BIOREACTOR SYSTEMS FOR PSCs EXPANSION AND DIFFERENTIATION
The bioreactor system provides expandable culture volume and dynamic physiological environment compared to conventional dish culture [78]. Ideally, bioreactor systems for PSC cultivation should meet several essential requirements, such as efficient mass transfer of oxygen and nutrients, waste transfer, and minimum shear stress [79]. These microenvironmental factors may affect cell pluripotency and fate during differentiation. Depending on the utilization, optimal PSCs expansion or differentiation can be conducted under various conditions (Table 2).
Generally, in the culture systems, PSCs are incubated at 37°C and pH 7.4. The instability of these culture conditions can inhibit cell proliferation, reduce pluripotency, and decrease cell viability [80, 81]. One of the main limiting factors in PSCs culture is the low pH which is mostly caused by the accumulation of secreted lactate in culture medium [45, 82, 83]. This condition occurred because of it`s higher glycolysis rate and cell cycle profile when compared to adult somatic cells, impacted to higher lactate secretion than glucose consumption [82, 84]. Therefore, the continuous removal of lactate is necessarily required in PSCs culture system.
Viscosity is correlated with fluid movement in culture systems. Appropriate medium viscosity properties are required to maintain the proper PSCs culture. Medium with lower viscosity exhibits more rapid mass transfer and uniform conditions in mass production. In contrast, a previous study showed that human PSCs culture in less viscous media may cause cell agglomeration and shear-induced apoptosis, leading to lower yields [8]. In addition, a recent study showed that the culture medium which exhibit continuous viscoelasticity by using an optimized non-toxic polymer, such as low acyl gellan gum can form large numbers of uniformly sized aggregates and maintain cells in a suspension without agitation, is one promising culture strategy [85]. However, scaling up of this method results in the limited mass transfer of oxygen and nutrients.
Culture Systems | Ease of Scale Up | Ease of Monitoring | Ease of Harvesting | Mass Transfer | Shear Stress | References of Application in PSCs Expansion | References of Application in PSCs Differentiation Types | ||||
---|---|---|---|---|---|---|---|---|---|---|---|
General culture systems | Conventional static culture | low* | low* | high | low* | low | hiPSCs[52,148,149], hESCs[52], miPSCs[150], mESCs[151] | Hepatocyte [152], chondrocytes [153], muscle fiber cells [154], lung and thyroid progenitor cells [155], odontoblast [156], cardiomyocytes [157] |
|||
Automation | medium | high | high | low* | low | hiPSCs [106,110,158-160], hESCs [161], mESCs [160], miPSCs [162] |
Cardiomyocyte [159], neural cells [160] |
||||
Stirred bioreactor | high | high | high | high | high* | hiPSCs [97,113,114,148,163,164], hESCs [97,114], miPSCs [165] | Hepatocyte [123], cardiomyocytes [122,166,167], pancreatic β cells [168], Endothelial cells [169] |
||||
Rotary bottle | medium | medium | high | high | medium | mESCs [170], hESCs [171] | Cardiomyocyte [127][166], osteogenic cells [170] | ||||
Hollow fiber bioreactor | medium | low* | low* | medium | low | mESCs [172], hESCs [131] | Hepatocyte [129,130,173] | ||||
Other culture techniques | Microcarrier | high | low* | low* | medium | high* | mESCs [174], hESCs [175], hiPSCs [175] |
Neural progenitor [176], endoderm progeny [177], hematopoetic cells [178], hepatocytes [179], cardiomyocytes [168,180,181]. | |||
Cell encapsulation | medium | low* | low* | medium | low | miPSCs [145], mESCs [142,146], hESCs [141,182] | Cardiomyocyte [143], pancreatic cells [183], definitive endoderm [182], osteogenic cells [170] |
Oxygen is one of the most important components in cell culture systems and it is involved in nearly all cellular aerobic metabolic cycles. In PSCs propagation, the concentration of oxygen in the culture media is a crucial factor in pluripotency and proliferation that maintains their cellular characteristics [86]. An in vitro study clearly demonstrated that differentiation was significantly reduced in human ESCs under hypoxic culture conditions (1-5% O2) compared to its culture under normoxic conditions (21% O2) by morphologically distinct growth area examination and expression of pluripotency markers according to biochemical and immunohistology assays [84, 87]. Another study showed that hypoxia of iPSCs cultivated under 5% O2 improved the efficiency of iPSC proliferation and enhanced the expression levels of transcription factors for iPSC reprogramming (Oct3/4 and Nanog), which are required to maintain pluripotency [88]. Repeated passaging of hESCs under normoxic culture conditions resulted in an increased number of differentiated cells and reduced self-renewal [89]. ESC growth under hypoxic conditions can preserve the pluripotency during repeated passaging and able to promote the formation of embryoid bodies after random differentiation [87]. Forsyth et al. reported that physiologic oxygen (2%) may reduce chromosomal damage and induce recovery after oxidative damage post room oxygen (20%) treatment [90]. These studies clearly showed that oxygen concentration affects PSC growth, differentiation, and EB formation. In this regards, the PSCs can be maintained or expanded in hypoxia condition and it can gradually switch into normoxia condition in a certain stage when the differentiation was performed. For example, the different oxygen concentration can be applied in hepatic differentiation by mimicking the original liver development during embryogenesis [91]
Cell aggregation control is very important for maintaining PSCs in an undifferentiated state and preserving cellular viability. In suspension hPSC propagation in vitro, cell-cell interactions are essentially required. Therefore, PSCs must grow in appropriate-sized aggregates. A recent study demonstrated that excess aggregation can direct PSCs into spontaneous differentiation, which occurred as the size of PSCs aggregates increased [92-95]. Moreover, insufficient oxygen and nutrition exposure can result in necrotic cells in the center of the aggregates [96]. Some approaches for controlling aggregation in suspension culture have been reported. Culturing in a microwell is one of the most widely used technologies for controlling aggregation. Seeded cells are divided and form a single aggregate in each microwell, resulting in the reproducible growth of uniform aggregates with high efficiency. However, the culture operations are still difficult to control because the high medium flow can be resulted from strong flow during culture medium changes, causing aggregates to detach from the microwell and fused each other. Therefore, it is technically required gentle treatment during medium removal or addition. Alternatively, to control these aggregations in suspension culture, dynamic conditions can be applied in the bioreactor system by adding impeller agitation of the culture media, but as an adverse effect, excessively high shear stress causes cell death and spontaneous differentiation [97, 98]. One of recently developed method for controlling aggregation is the addition of lipid-rich albumins, such as Albumax. This compound can reduce aggregation by inhibiting E-cadherin-mediated cell-cell attachment. A previous study showed that 0.2-0.5% Albumaxin the culture medium is the optimal concentration for obtaining appropriately sized aggregates and higher hiPSC yields in 12-well plates in well-preserved pluripotency [99].
Shear stress is a particularly important biomechanical factor in mammalian cell culture. Most of the cell types respond to shear stress by physiological or gene expression alterations [100, 101]. Generally, excess hydrodynamic stress by vigorous agitation can decrease the viability of mammalian cells [76, 102]. In addition, excess agitation during cultivation may affect the phenotype and characteristics of PSCs. For example, an excessive centrifugal force of 1000 ×g or more may induce phenotypical shifting and decrease the proliferation rate during expansion [80]. A study conducted by Sargent et al. revealed that exposure to hydrodynamic stress conditions not only significantly affected aggregates formation and structure, but also induced downregulation of pluripotency genes, resulting in spontaneous differentiation [103]. Moreover, in the suspension culture systems, cells in aggregates are exposed to higher shear stress than individual cells because of their large particle diameter [104].
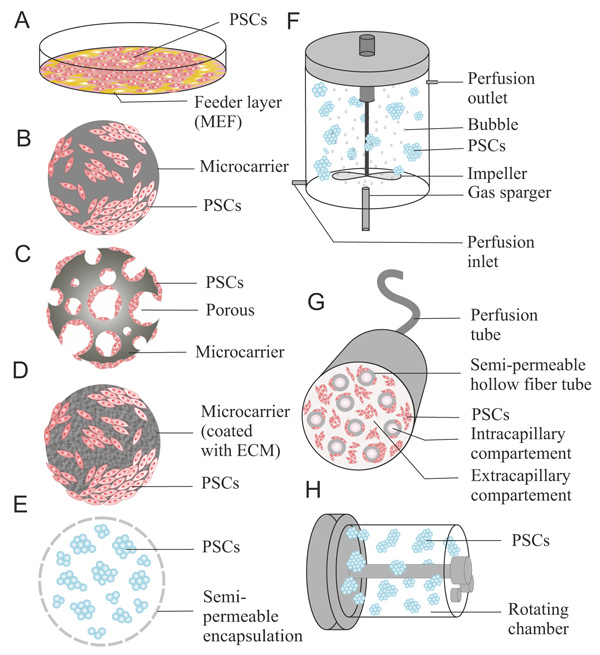
5. CULTURE SYSTEMS IN PSC MASS PRODUCTION
5.1. PSC Adhesion Culture Systems
Conventionally, PSCs are cultured in tissue culture dishes (Fig. 1A), multiwell plates, and tissue culture flasks. These methods provide two-dimensional surfaces for the growth of PSCs. Under these two-dimensional conditions, morphological examination of PSCs in colonies or as single cells can be more easily observed by direct visualization using a microscope, which is important for monitoring both undifferentiated PSCs or changes in PSC shape during various stages of differentiation. These disposable systems are also relatively simple, easy to handle, and inexpensive. However, in large-scale PSC production, this system represents several disadvantages, such as a limited surface area (approximately 2-225 cm2 working area), which is required for adherent cells. This condition makes the scaling up difficult. Furthermore, the static conditions of the culture can cause poor mixing of the medium, leading to concentration gradients in PSCs culture [38]. Because of these gradients, controlling parameters such as temperature, pH, and dissolved O2 is challenging in adhesion culture systems. Multiple plates or flasks (or flasks with multiple trays) are required to obtain large numbers of cells simultaneously, but these vessels also require repeated extensive handling for feeding and subculturing, making the process more laborious and prone to contamination [105]. In addition, the considerable amount of these disposable plastic materials waste can cause an environmental problem.
To overcome the difficulty of handling, an automated culture system has been developed by utilizing a machine, robotics, and programming to conduct cell propagation. Automation systems show many benefits, such as the ability to significantly reduce the working time for the technician, provide automated and scheduled screening, imaging, or cell maintenance based on cell growth, and minimize the risk of contamination [106, 107]. Most of the commercially available automated culture systems, such as Cytogration™ systems, SelecT™ systems, and AcCelerator™ systems, are based on continuous cell maintenance, which automatically delivers media containing nutrients and removes cellular waste products [108]. They also allow for periodic automated evaluation, such as to detect the pH, nutrition and waste concentrations, cell growth rate, and cellular viability [109]. These systems provide more consistent data regarding the culture procedure [109]. Soares et al. established a protocol for maintaining hiPSCs using feeder-free and chemically defined media on the inCompacTSelecT™ automation platform without significantly decreasing pluripotent capabilities [110]. Current automated culture technology, such as CellCelector also equipped with cell sorting combined with a cell scrapping module to selectively harvest the PSCs colony from feeder cells [111].
5.2. PSC Suspension Culture Systems
5.2.1. Stirred Bioreactor
A stirred bioreactor is one of the most common types of bioreactor systems used to cultivate PSCs on a large scale. This culture system provides a homogeneous environment for the expansion of ESCs and iPSCs by continuous agitation in large working volumes (50 mL-200 L) [5, 112, 113] (Fig. 1F). Various types of feeding operations such as batch, fed-batch, or perfusion have been developed. In these culture systems, PSCs are cultured as aggregates or adherent colonies combined with various 3D cell culture technology, such as microcarrier, cell encapsulation, or scaffold. The combination of culture techniques may enhance scalability, improve oxygen and mass transfer, and ease complicated handling. In addition, this bioreactor is controllable by installing sensors to detect pH, dissolved O2, medium level, and temperature [114].
The stirred bioreactor mainly using an agitation mechanism with an impeller or stirrer to obtain a uniform culture mixture. In suspension culture, this agitation is important for maintaining pluripotency. A previous study evaluated various levels of shear in a stirred suspension bioreactor to determine its ability to maintain aggregate size. The results showed that the higher level of shear force in the suspension bioreactor produced smaller hESC and hiPSC aggregates compared to lower shear force [114]. On the other hand, excessive mechanical agitation can produce high shear stress, which can harm PSCs by affecting their pluripotency and viability [114-117]. Such damage can be relieved by decreasing the rotational speed, modifying the shape and size of the impeller, or by adding additional substances, such as bovine serum albumin, to protect the cells from shear stress.
In some bioreactors, installation of a gas sparger can accelerate gas transfer and increase hydrodynamic movement. However, the bubbles produced by the gas sparger may potentially harm the cells. These bubbles rise faster than the medium flow and slowly carry the cells to the surface, increasing the shear stress to the cell membrane [118]. This condition was previously demonstrated by microfluidic assessment, which mimics bubble-induced shear stress, in Chinese hamster ovary cells [119]. In addition, bubble accumulation can form a foam, causing bubble burst-associated damage at the medium surface [120]. In most cases of mammalian cell culture, small bubbles (approximately <2 mm in diameter) are more significantly damaging to cells than large bubbles (10–20 mm). Agitation by an impeller can also enhance cell membrane damage by disrupting the large gas bubbles arising from the sparger [121].
Stirred bioreactor also can be applied in PSC differentiation. Although one study demonstrated its capability to support the high-scale differentiation of PSCs such as cardiomyocytes from murine ESCs [122] and hepatocytes from hiPSCs [123], the hydrodynamic conditions in this bioreactor appear to produce a high subpopulation of cells with heterogeneous phenotypes of various types of cells [123, 124].
5.2.2. Rotary Bottle Culture Systems
Rotating bottle culture systems enhance the efficiency of aggregate formation, increase cell yield and homogeneity, and do not adversely affect differentiation [103]. This culture system was first developed by NASA to investigate cell culture systems in the space. Rotary culture systems consist of rotating 3D chambers to accommodate the cell culture, which is suspended under microgravity conditions, creating a continuous homogenous environment with low shear stress for PSCs culture (Fig. 1H). These hydrodynamic properties are very important for supporting the maintenance of aggregate formation in suspension culture. This system improves the homogeneous condition and prevents excess PSCsagglomeration [103, 125]. In large-scale production of PSCs, their systems complexity causes difficulties in continuous feeding and uses a low working volume (approximately 10–500 mL) [112].
Several studies have demonstrated that the dynamic rotary suspension culture enhances PSCs aggregation and differentiation by supporting specific signaling pathways involved in directing cell fate. Lei et al. found that the biomechanical properties of this culture system induce Wnt/β-catenin signaling, which is responsible for mouse ESC differentiation into the mesoderm and endoderm [126]. Another study revealed that the cardiogenic differentiation of murine ESC aggregates in a rotary culture environment can produce a higher percentage of contracting cardiomyocytes compared to differentiation in adherent culture [127].
5.2.3. Hollow Fiber Bioreactor
The hollow fiber bioreactor is a culture system based on a perfusion system that uses small semi-permeable membrane hollowed fiber tubes. By circulating the culture medium, this system can provide an exchange between small molecule components, such as nutrients and metabolic wastes (Fig. 1G). This system mimics the microenvironment of blood vessels in vivo by using two different compartments. The first compartment is an intercapillary space within the hollow fibers, while the second is extra-capillary space surrounding the hollow fibers [79]. The cells are cultured in the extra-capillary space, and oxygenated medium at an appropriate pH is circulated through hundreds to thousands of capillaries within the hollow-fiber cartridge using perfusion systems. Oxygenation and pH control is achieved by a gas-permeable surface in a CO2 incubator. The exchange of nutrients and waste products is achieved by diffusion through the semi-permeable membrane fibers. A separate culture compartment and perfusion compartment produces the low-stress environment required for maintaining PSC pluripotency and viability. In this system, the culture compartment can be filled with up to 108 cells/mL (near the tissue density in vivo) [128]. Therefore, this system requires less medium and fewer growth factors than traditional culture methods. This high-density culture also can facilitate the three-dimensional growth resembling the in vivo tissue structure to support PSC differentiation. Recent studies showed that the functional activity and formation of several tissue-like structures were increased in hepatocyte-derived hiPSC aggre-gates after hepatic differentiation using this system [129, 130].
However, hollow fiber systems have some disadvantages, such as difficulties in monitoring and controls. At high cell densities, spatial concentration gradients in the extra-capillary compartment can form, based on the distance of the PSCs to the intercapillary compartment. Previously, a study by Roberts et al. evaluated the potential and feasibility of using hollow fiber systems for hESC mass production. The results indicated that hESCs can be scaled up in a hollow fiber bioreactor without a significant decrease in pluripotency [131].
6. OTHER IMPROVEMENTS TO ENHANCE PSC CULTURE SYSTEMS
6.1. Microcarriers
In optimizing the scalable culture systems for expanding undifferentiated PSC culture, traditional adherent cell culture can be utilized on polymer- or hydrogel-based microcarrier beads [132]. Microcarriers can provide a high surface area for cell attachment and proliferation. This technique enables adherent-type cells to be treated as similar to the suspension culture. Compared to conventional static adherent culture, the main advantage of microcarriers is that they can accommodate a larger number of cells and occupy less space in the bioreactor [133]. According to a previous study, culturing on microcarriers was conducted using 2- to 4-fold higher densities of iPSCs than in conventional adherent culture [134].
Generally, microcarriers are divided into two types: nonporous microcarriers and porous microcarriers (Fig. 1B, 1C). Solid-filled microspheres or nonporous microcarriers are composed of bioceramic or biopolymers. Anchorage-dependent animal cells can be grown on the surface of small-diameter spheres that can be maintained similarly to in suspension cultures. Equal effects of nutrients and growth on the surface of microcarriers can be achieved to expand cells with homogeneous characteristics and differentiation. However, direct contact between the attached cell and media caused some frictional and shear stress, which can damage the cells, mainly when combined with highly hydrodynamic conditions such as in a stirred bioreactor. Porous microcarriers contain pores and channels that are large enough for cells to enter and grow within the carriers, which allow for culturing at higher cell densities than for nonporous microcarriers. In addition, these microcarriers can create a microenvironment inside the beads, protecting the cells from shear and frictional stresses caused by aeration and agitation in a dynamic bioreactor. Thus, porous microcarriers are more appropriate for large-scale mass production of cells compared to nonporous microcarriers. However, it is difficult to observe cells inside the microcarriers and separating and collecting the cells is challenging. In addition, the gradients of conditions, such as different in hydrodynamic properties, cell-cell interactions, and nutrition or oxygen concentrations, have been observed in pores. Consequently, nutrient transfer and cellular waste product release will be more limited for the cells inside the pores [135, 136]. This condition also can cause variability among cultured PSCs undergoing expansion or differentiation.
Nonporous microcarriers can be coated with ECM such as collagen or fibronectin to enhance cell attachment and adhesion (Fig. 1D) [132, 137]. These coatings are completely dissolvable in trypsin solution, which allows the sampling, counting, and harvesting of the cells without interference from the carriers. Chen et al. investigated the cellular attachment efficiency, growth, and pluripotency of hESCs using 10 types of microcarriers. The study revealed that poor growth and gradual loss of pluripotency occurred at the same time because of a lack of ECM interactions in uncoated microcarriers. Higher yields and stable pluripotent states of hESCs grown using Matrigel-coated microcarriers were achieved compared to using other types of microcarrier culture [138]. A study also reported the successful differentiation of the mesoderm from hESCs and hESC using similar types of microcarriers [139].
Although cellulose is the most common material used for microcarriers, various other materials have also been used, such as hydroxyapatite, tricalcium phosphate, and glass ceramics. Synthetic degradable polymers such as polylactic acid, polyglycolic acid, and polycaprolactone are also used as microcarriers. According to a previous study, hESCs did not attach and grow on microcarriers with a negatively charged surface [65]. The sizes of the spheres are diverse depending on the conditions, such as material content, surfactant type and amount, type of solvent, and stirring speed. Synthetic polymers typically lack hydrophilic and adhesive ligands, which are required for cell attachment. Therefore, the surface requires hydrophilization and coating with adhesive proteins such as Matrigel, fibronectin, or collagen. For PSCs propagation, it has been reported that the use of combined materials in microcarriers can affect their cellular characteristics. For example, combining hydroxyapatite powder within polylactic acid can potentially improve cell adhesion, cell growth, and osteoblastic differentiation [135].
6.2. Cell Encapsulation
The main principle of cell encapsulation is to envelope viable cells or aggregates within semi-permeable membrane layers to protect the cells from shear stress and to accumulate the native products secreted from cells while exchanging nutrients and wastes (Fig. 1E) [140]. Various sizes of capsules are available for cell encapsulation, which are 0.3-1.5 mm or larger. Enclosing PSCsin hydrogel can create a microenvironment with cell-hydrogel contact and auto/paracrine effects while protecting them from the hydrodynamic shear stress. A report showed that encapsulation provided more than 6 months of prolonged feeder-free PSCs expansion in well maintained pluripotency [141]. Another report showed that the differentiation markers in encapsulated PSCs were downregulated compared to cells in conventional suspension culture, which prevented unexpected PSCs differentiation [142]. Besides for PSCs expansion, the cell encapsulation can also be utilized for differentiation. Kerscher et al. performed the cardiac differentiation of hiPSCs by using direct hydrogel encapsulation [143]. Various types of hydrogels are used for encapsulation, such as PEG [143], agarose [38], hyaluronic acid [144], and calcium alginate [145]. Calcium alginate hydrogel is the most widely used hydrogel for encapsulation because of its fast and reversible gelation. A recent investigation revealed that alginate composition can influence the cellular phenotype of murine ESCs towards the preservation of pluripotency or differentiation [146].
In contrast to these advantages, the application involves an encapsulation and decapsulation process, which is challenging when using hydrogel with non-reversible gelation. In addition, the PSCs which exhibit a high proliferation rate can escape from hydrogel capsules and break outside the capsules when it could not accommodate the cell growth. Coating capsules with a solid shell can prevent this escape but may increase the difficulties of decapsulation [145]. Thus, cell encapsulation can be utilized for long-term maintenance or implantation to achieve immuno-isolation by protecting cells from antibody recognition.
6.3. Perfusion Systems
To optimize the mass transfer of essential compounds, some bioreactors are equipped with continuous perfusion systems. This configuration provides better oxygen transport and enhance the cell feeding by frequently removing byproducts or metabolic wastes and replacing the fluids with fresh medium containing nutrients [98]. One study reported that PSCs cultivation successfully maintained with good viability, pluripotency, and genetic stability after 6 days of culture by automated perfusion feeding of mESCs in a Petri dish. This system also significantly enhanced the cellular growth rate [147].
CONCLUSION AND FUTURE DIRECTIONS
As described above, various bioreactor systems provide different microenvironments and physiological factors. In addition, the optimum condition for PSCs depends on the stage of culture, such as maintenance, propagation, or differentiation into target cells. For example, maintenance and propagation require rapid exchange of nutrients and wastes because of the rapids anaerobic respiration in these cells. Therefore, the perfusion system or fed-batch culture system is preferred for PSCs propagation. In contrast, the culture costs for differentiation are much higher than those during maintenance because it requires numerous amount of growth factors combinations. Therefore, the use of growth factors should be reduced, such as in dialysis culture systems. Thus, choosing an appropriate culture system is important for the industrial application of PSCs.
CONSENT FOR PUBLICATION
Not applicable.
CONFLICT OF INTEREST
The authors declare that there is no conflict of interest, financial or otherwise.
ACKNOWLEDGEMENTS
Declared none.