All published articles of this journal are available on ScienceDirect.
Evaluation of the Fatigue Performance and Degradability of Resorbable PLDLLA-TMC Osteofixations
Abstract
The fatigue performance of explanted in-situ degraded osteofixations/osteosyntheses, fabricated from poly (70L-lactide-co-24DL-lactide-6-trimethylane-carbonate or PLDLLA-TMC) copolymer was compared to that of virgin products. The fatigue test was performed on 21 explants retrieved from 12 women and 6 men; 16-46 years by a custom-designed three-point bend apparatus using a staircase method and a specified failure criterion (an increase of the deflection of the specimen > 1 mm) with run-out designated as “no failure” after 150,000 loading cycles. While all the virgin products showed run-out at 38N, all of the specimens fabricated from explants failed at this load level. For the explant specimens, although there was a trend of decreased failure load with increased in-situ time, this decrease was pronounced after 4 months in-situ, however, not yet statistically significant, while a 6-month in-situ explant had significantly less failure load. Three and four month in-situ explants had highly significant differences in failure load between measurements close and distant to the osteotomy line: p=0.0017 (the region of maximum load in-situ). In the virgin products, there were only traces of melt joining and cooling, left from a stage in the manufacturing process. For the implants retrieved after 4.5 months in-situ, the fracture surfaces showed signs of degradation of the implants, possibly caused by hydrolysis, and for those retrieved after 9 months in-situ, there were cracks and pores. Thus, the morphological results are consistent with those obtained in the fatigue test. The present results suggest that resorbable osteofixations fabricated from PLDLLA-TMC are stable enough to allow loading of the healing bone and degrade reliably
1 . INTRODUCTION
Patients with complex fracture patterns receive internal fixation with increasing frequency, as these cannot be stabilized sufficiently with conservative treatment means, nor do displaced bone structures remodel into functional anatomy (e.g. at the mandibular angle and condyle) [1, 2]. Stainless steel and titanium miniplate osteofixation, or commonly called “titanium-osteosyntheses”, have several drawbacks, such as potential growth disturbance, passive migration, palpability and corrosion. Metallic debris, arising from corrosion, enters local lymphatic nodules, causing local irritation, dysesthesia, temperature sensitivity, pain, infection, and interference with diagnostic and therapeutic radiation [3,4]. Bone weakening has been observed, and this is caused by local stress-shielding of rigid mandibular and extremity plate osteofixations [3,5,6]. This is why operative removal in a second operation is necessary and recommended. A second operation can be avoided, however, by using resorbable osteofixations.
Given sufficient stability, a resorbable osteofixation increases the load placed on healing bone through progressive degradation [4,7-9]. Complete disintegration is sought after 3 months of stable fixation and should cause no local irritation of tissues [9,10]. The biodegradation of polymers used in resorbable osteofixations has been demonstrated in many laboratory in-vitro and in-vivo animal studies [11-16], but only few in-situ patient studies have been reported [17,18]. These latter studies have some limitations, such as small number of explants and the results being applicable only to the specific polymer used for fabricating the osteosyntheses. This suggests that degradation studies need to be conducted on a polymer-by-polymer basis [9,10,19-21].
Studies of crystalline poly(L-lactide) (PLLA) have shown, that molecular weight, crystallinity, and melting point remained constant after 2 years in vitro, indicating slow, if not incomplete degradation [19,21]. Crystalline polyglycolide (PGA) has been reported to degrade significantly faster than PLLA [19]. Both of these polymers have been implicated in clinically apparent foreign-body reactions in patients [22], but not in animals [22]. In-vitro (laboratory experimentation) or in-vivo (laboratory animals) results showed limited transferability to the in-situ that means the “in-patient” situation. For example, test protocols for an accelerated in-vitro “aging” of the implants can result in degradation pattern that are not representative for the in-situ situation (Landes / Jaeger, unpublished results) Copolymers of PLLA with PGA (PLGA) combine the benefits of both materials — that is, the adequate strength retention of PLLA and the relatively fast degradation rate of PGA. In a previous report we showed that in patients, implants fabricated from a 85%mole:15%mole PLGA copolymer degrade considerably faster in-situ, than those fabricated from a 70%mole:30% mole P(L/DL)LA (racemic mix of L and DL lactide) copolymer. Both copolymers degrade reliably in-situ, mainly by hydrolysis, but at a rate that is slower than what occurs in-vitro [18]. The exact moment of total resorption can only be extrapolated from the degradation data of explants and the macroscopic absence of material in patients on surgical revision [18]. Three point bending test (explants were too small) and scanning electron microscopy were not performed at that time.
In the present work, we investigated the fatigue performance and degradation characteristics of a new generation of resorbable osteofixation, fabricated from a new copolymer, poly(70%moleL-lactide-co-24%moleDL-lactide)-6%mole trimethylane-carbonate (PLDLLA-TMC) in patients. This polymer has the advantage to be less brittle in handling, due to the addition of TMC. In 2.5 mm strength, PLDLLA-TMC is also more stable than other available polymer osteofixations [11,15]. PLDLLA-6TMC so far has only been investigated for degradation in-vitro (laboratory experimentation) and in-vivo (animals), not in-situ (patients) [11,15].
2 . PATIENTS AND METHODS
2.1 . Patients
Eighteen patients (12 women and 6 men) participated in this study and signed a detailed document of informed consent. Only patients who accepted the possible risks were included. Furthermore, our Institutional Review Board / Board of Ethics approved the protocol that we used to analyze the material, which was removed in the course of a secondary operation for local access to osteotomy of bone, e.g. in rhinoplasty or for augmentation. The patients were aged 16 to 46 years (24 years on average), and the primary operations (i.e. the implant placements) were carried out between April 2005 and April 2007. Treatment of patients was per ISO 9001:2000 and revised guidelines in the maxillofacial and plastic facial surgery unit.
2.2 . Implants
Within the total patient population, eighty patients had received a racemic random mix of Poly-70L mole%/24D Lmole%-lactide with 6mole% Trimethylenecarbonat (PLD-LLA-TMC; INION CPS®, INION OY, Tampere, Finland) 2.0mm system osteosyntheses, retaining maxillary or mandible fractures and osteotomies. Only 26 of the cases had secondary surgery as septorhinoplasty, chin plasty, alveolar process augmentation and dental implant insertion and required explantation during the secondary procedure.
All implanted material was FDA / CE / TÜV approved and certified (obligatory product marks for the US, European, and German markets, respectively, indicating compliance/certification with requirements specified in the American Consumer Safety Act, European Marketing Directive, and German Marketing Directive). Screws and plates were injection-molded (Fig. 1). As screws attached the plates to the bone and plates unite the fragments, screws and plates were always applied together in patients.
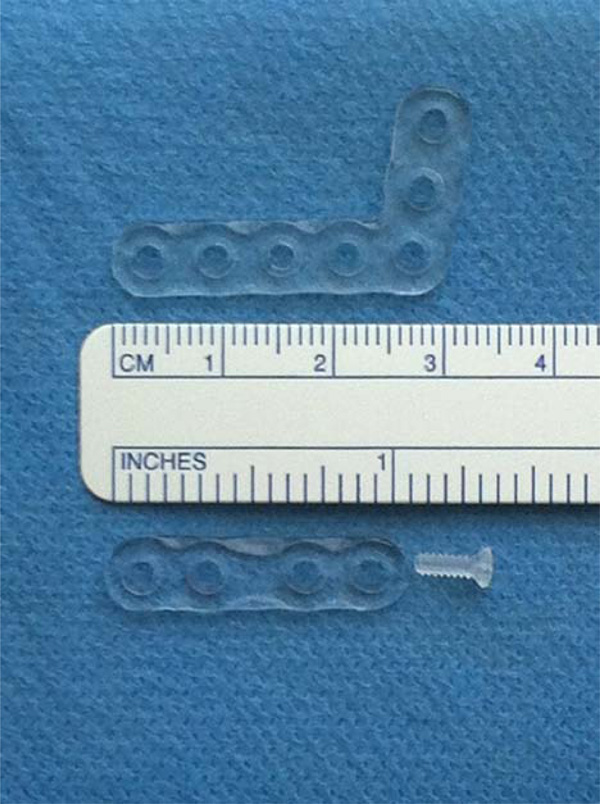
Shows the 7-hole-L-plate evaluated within this study and straight 4-hole-plate in 2.0mm strength, moreover a 2.0x6 mm screw.
The straight 2.0 mm system plate as the 2.5 mm system plates and screws were not biomechanically evaluated in this report.
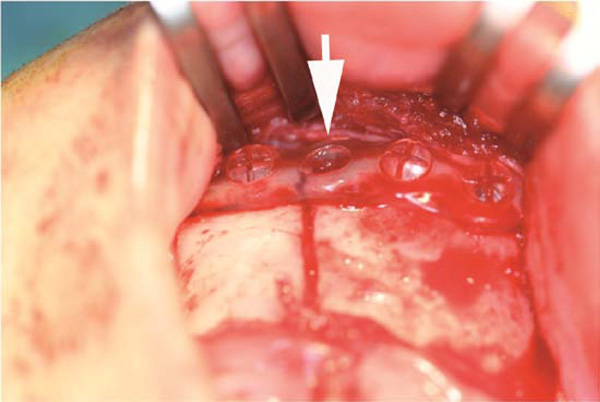
Photograph of a PLDLLA-TMC (arrow) osteofixation in a mandible fracture case.
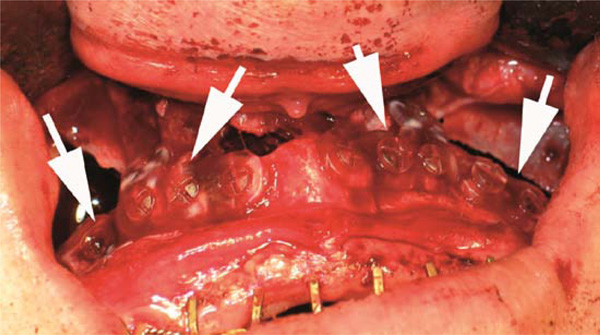
Photograph of maxillary osteosyntheses after Le Fort I advancement (arrows).
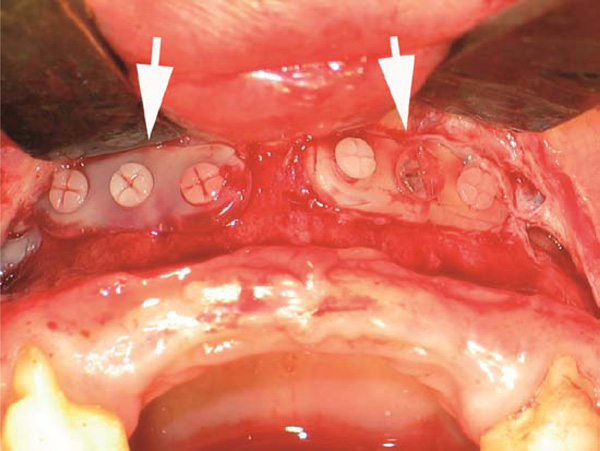
Photograph of the identical osteofixations (arrows) after three months at the secondary surgery of alveolar process augmentation. Due to the incipient hydrolysis the screws and plates appear opaque.
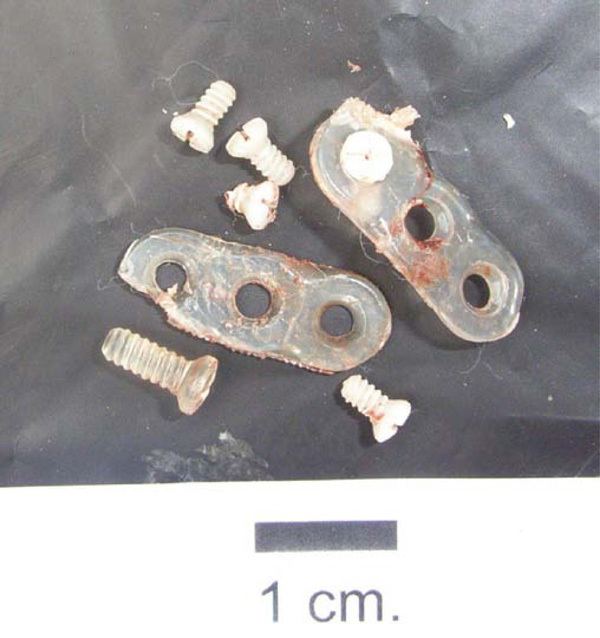
Photograph of “L-shape-plate” explants after 3 months in-situ, where the injection-molded screws of 2.0mm diameter show more opacity. Size of the implant and material composition affect both degradation behavior, the latter can be ruled-out as the polymer is identical. Moreover at the inferior left corner, a 2.5 mm-system screw shows lower opacity probably due to her bigger diameter and volume that per-mits only slower hydrolysis as in the 2.0 mm screws.
Upon removal the L-plates were cut at their knee, as such three-hole fragments could easily be positioned in the fatigue test set-up.
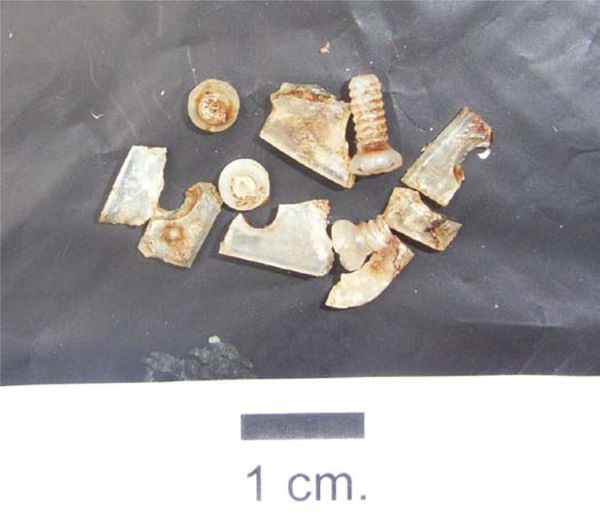
Photographs of explants evincing progression of opacity as a sign of hydrolysis within the implant after 5 months, also affecting the plate fragments at this stage. Three-point bending testing beyond 6 months in-situ was impossible, as explants broke too easily into small pieces upon removal or handling. The resulting fragments could no longer become fitted into the fatigue test set-up.
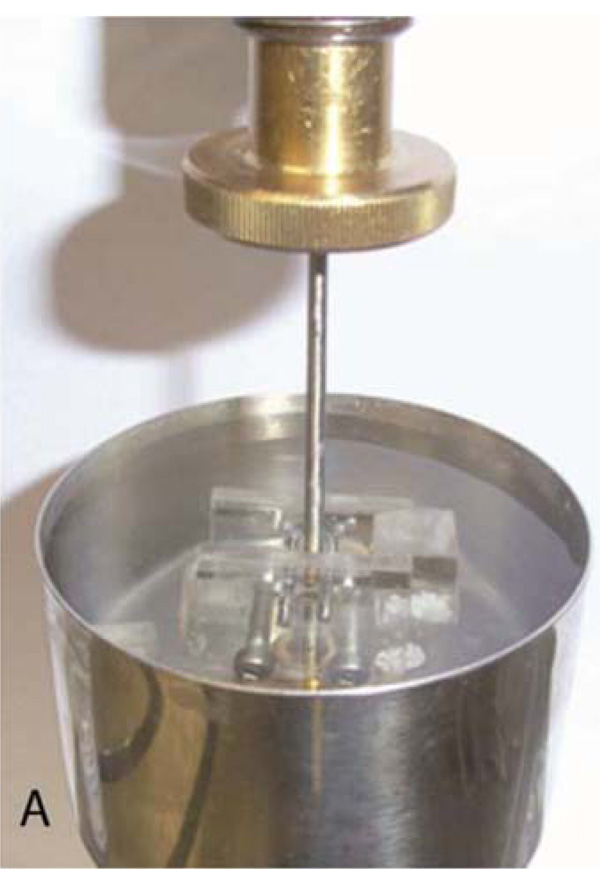
Photograph of an explant specimen positioned in the three-point bending test set-up. The mechanical tests were carried out at room temperature, the explants were conditioned in deminer-alized water at 37°C for one week.
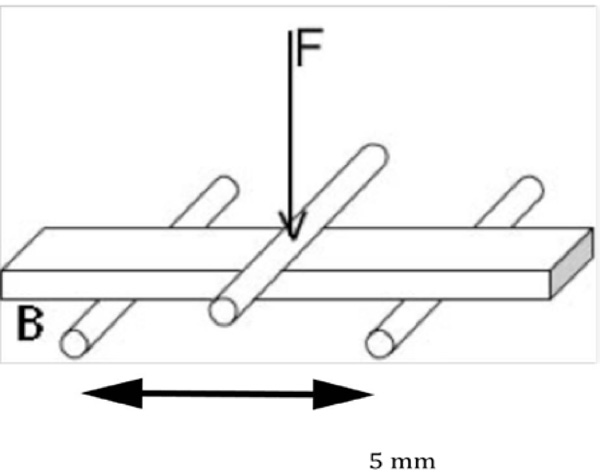
Sketch of a 3-point-bending test set-up.
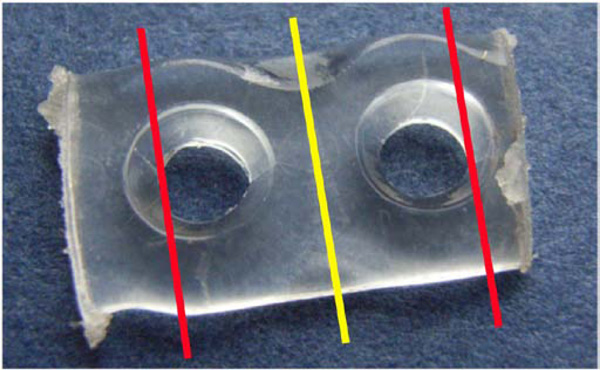
Specimen for 3-point-bending experiments. The upper loading roller exerted the pulsatile sinusoidal force in the center between two holes (yellow line). The specimen rested on the lower loading rollers at the positions marked with red lines.
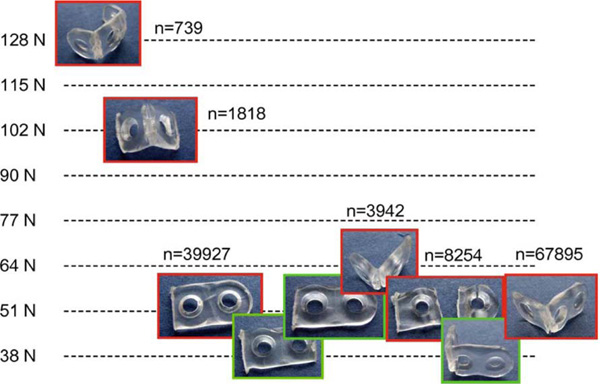
Results of the fatigue tests on virgin products with a run-out level of 38 N, MFL: 49 N ± 11 N. This means at 38 N all out-of-package specimens survived 150,000 load cycles, at loads above 38 N failures occurred.
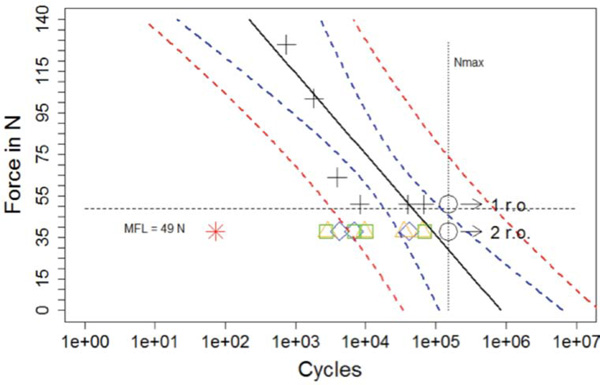
Results of the fatigue tests on virgin products (+: failures, О: run-outs) and explanted implants (all failures; *:6 months in-situ, ⋄: 4 months in-situ, r∆: 3 months in-situ, □: 2.5 months in-situ).
All explants did fail at the F = 38 N level and the implantation period correlates with reduction in life-time within the fatigue strength exami-nation.
Solid line: regression line including +, О, blue dashed line: 95% confidence interval for the regression line, red dashed line: 95% prediction interval, i.e. an additional measurement at a force level will lie with 95% probability within this prediction interval. The result for the implant 6 months in situ (*) lies outside of the prediction interval, as a result the degradation resulted in a significant decrease of its fatigue strength
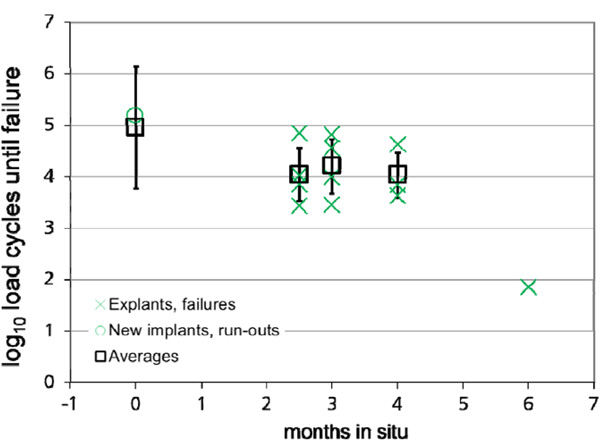
Results of the fatigue tests on virgin products and explanted implants (green symbols), carried out at 38 N related to the in-situ time
A moderate drop of the lifetime occurs between 2.5 - 4 months, a significant drop in strength after 6 months. For fractures of the explants, the mean values and standard deviation are plotted. For the run-out level of the new implants, the results of the linear regression with F = 38 N and the "wide"confidence interval are shown instead of the mean value and the standard deviation
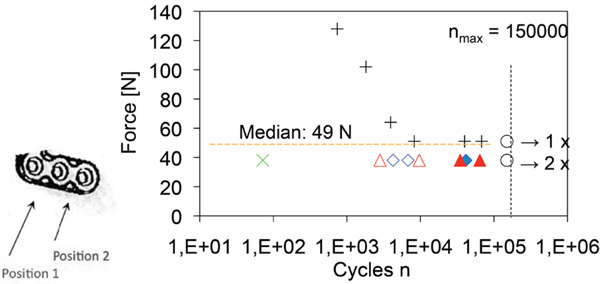
Results of the fatigue tests on virgin products (+: failures, О: run-outs) and explanted implants x: 6 in situ months, ⋄: in situ 4 months, ∆: in situ 3 months: influence of testing position on explants from which test specimens were taken. Open symbols: position 1, closed symbols: position 2. A t-test of 3 / 4 months in-situ explants measured at position 1 versus position 2 showed highly significant results (p=0.0017).
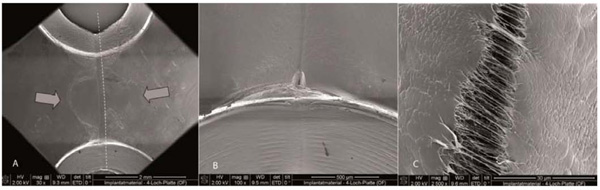
Fig. (8a). Morphology of the surface of a virgin implant, revealing a smooth appearance and traces of the processing as joining of the melt during injection molding.
Fig. (8b). Is a new implant showing a local crack at the interface to the screwhole from the manufacturing process.
Fig. (8c). A local crack at the interface of a virgin product, possibly caused by residual stresses after cooling. The position of the crack is most likely uncritical (within neutral fiber).
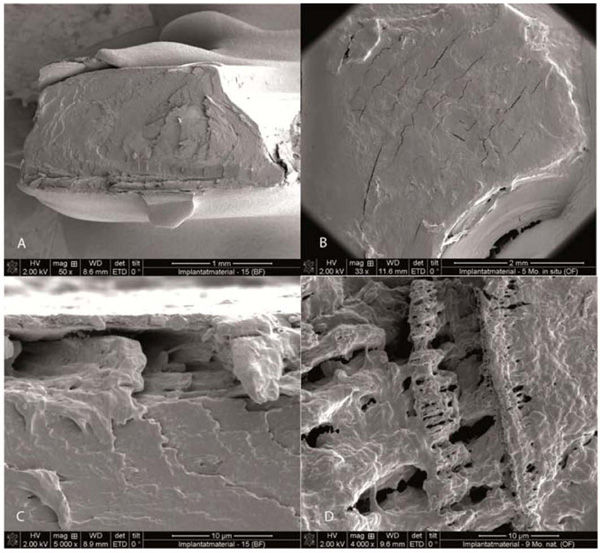
Fig. (9a). Fracture surface of a fragmented implant explanted after 4.5 months in situ (X 50).
Fig. (9b). Fracture surface of a fragmented implant explanted after 4.5 months in situ (X 5000).
Fig. (9c). Cavities form below the implant surface.
Fig. (9d). Fracture surface of a fragmented implant explanted after 9 months in situ, showing pores and cavities in the implant body, a consequence of hydrolysis.
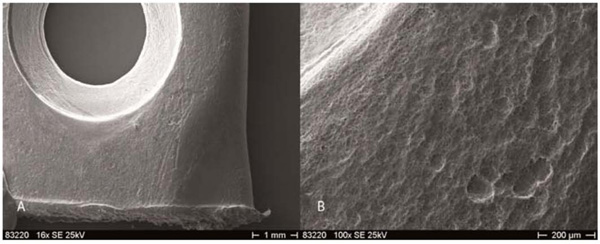
Fig. (10a). REM reveals in-vitro laboratory-aging associated surface roughness and dimples on the surface of the implants.
Fig. (10b). At bigger magnification these irregularities were contrasting the smooth surfaces of in-situ aged explants.
2.3 . Procedures
All osteofixations were performed using standard maxillary and mandibular procedures for osteotomies and fracture synthesis (Fig. 2, a-c). After subperiosteal exposure and segment repositioning in the maxilla, 4–8-hole 2.0 mm system plates were fixed to both segments with 2–4 screws of 2.0× 6 mm dimensions. In the mandible 2.5 mm system was used in identical fashion at the same operation date.
Intraoperatively immersion for 60 s in a basin containing sterile water, at 55 °C, rendered the plate flexible after 5-7 s in their glass-transition temperature interval (50–70°C), to allow for adaptation to the shape of the bone. Using the 2.0 mm-strength system, screw-holes were drilled using a 1.75 mm-diameter burr, the thread was cut using a tap of 2.0 mm diameter thread-to-thread, and the 2.0 mm-diameter screw (diameter thread to thread) was inserted. Plates were 2.25 mm thick and 6 mm wide. In the 2.5 mm strength system, the burs had a diameter of 2.25 mm, the tapper 2.5 mm thread-to-thread and the screw that was inserted also had a diameter of 2.5 mm.
2.4 . Explantations
All the patients underwent local biopsies and explanations of resorbable material at different times, Fig. (3, a, b), but only the 2.0 mm strength system L-shape-plates were analyzed: 21 explants retrieved from 12 women and 6 men; 16-46 y. The removal included local incision, dissection and exposure; then the implant was intentionally removed as much as needed for the augmentation or osteotomy. Therefore at times a fragment that was large enough to place in the bending apparatus was obtained. The harvested material was cleaned with water and dried with cotton swab before dry storage for use in laboratory examination.
After a minimum of 3 months in-situ, all the implants were intact before removal but broke readily during removal. The mechanical strain that occurred during explantation was caused by surgical isolation from the surrounding fibrous tissue, which can overstrain the residual bending strength of the plate. During surgery, when the complete implant and fibrous tissue were removed together, the implant broke when dissection from the surrounding tissue was ventured. Further exposure therefore could not be justified due to patient discomfort.
2.5 . Specimens
After removal of the explants from the patients, these specimens were stored dry without further loading, until the testing was performed.
In a previous report, we were unsuccessful in performing four-point bend tests on the removed implants, because either they were too small or had been bent during the primary procedure for clamping in the testing apparatus. [18]. In the present work, however, we were successful by using a modified smaller three-point bending apparatus and explants with a length above 8mm long. The set-up and testing was mainly performed to assess the fatigue performance of the explanted material and compare it to that of out-of package and laboratory-aged material (Fig. 4, a, b).
For-point-bending tests result in comparison with three point bending tests in general in a lower strength due to the higher loaded or “critical” volume, which is tested in a four-point-bending test. Since the aim of the study is a comparison of different implants which was carried out on test samples of comparable shape on the same three point bending arrangement, the results of the study are not affected. In addition, four-point-bending tests were not feasible for the size of the samples.
2.6 . Fatigue Testing
These tests were carried out using a stress amplitude that is lower than the yield strength of the implant material. In performing fatigue tests on osteosyntheses, two approaches may be taken. In the first, an osteofixation plate is attached with bone screws to a member whose properties are similar to those of cortical bone, and the plate is then loaded so that the stresses are the same as those seen physiologically. In the second approach, the fatigue performance of the implant material may be evaluated using a standard test set-up (three-point bending, tension-tension, compression-compression, etc.). Since mostly fragments of the explants were available in the present study, we utilized the second approach. Whereas the first approach gives some insight into the fatigue performance of an entire component (in this case the implant), the second approach yields some insight into the fatigue performance of the material. In our case, the latter approach is sufficient in order to assess the effect of the materials degradation on its fatigue performance.
The tests were carried out in demineralized water, at room temperature, using a commercially available servohydraulically-actuated universal materials testing machine (Mini-Bionix; MTS Systems GmbH, Berlin, Germany). The distance of the lower loading rollers of the three-point-bending set-up was 5 mm. A sinusoidal pulsating load (frequency = 5 Hz) starting value: F0 = 128 N, step width: ΔF = 13 N was applied in load control. The maximum number of load cycles (Nmax) that we used (150,000) is that typically used in fatigue testing of osteofixation plates used in cranio-maxillo-facial surgery [23]. Specimens which reached Nmax = 150,000 cycles without failure were taken out of the experiments and classified as “run-outs”. The peak value of the deflection of the test plate, after 500 loading cycles, was recorded. If this deflection exceeded 1 mm or if crack(s) were visible in the plate, the plate was considered to have failed.
The virgin (unimplanted) identical products used were 7-hole L-plates. They were cut to obtain specimens of the same size as the explanted implant fragments. The implants were cut such that a test specimen contained two holes. The upper loading roller exerted a force in the center of a strut connecting two holes. The implant rested on the two lower loading rollers, which were positioned near the center of the hole (Fig. 4c). The specimen dimensions (virgin implants and explants) were generally: length 8 mm (distance of the support rollers: 5 mm), height 1.27 mm), width: 6.8 mm (Fig. 4c).
Prior to testing, all the implants were soaked in water for 7 days in order to achieve an essentially complete saturation.
The testing was carried out using the staircase method given by Dixon and Mood. Fig. (5) [24, 25]. This involved selecting an initial load F0 (preferably in the range where lifetimes just below nmax occur) and load step (ΔF). If run-out occurs at F0, increase the load by ΔF; if, however, the specimen fails at F0, decrease the load by ΔF. The testing gathers fatigue data at stress levels, which lie in the vicinity of the Median Fatigue Limit (MFL). The MFL was calculated according to the staircase method of Dixon and Mood, see references [24,25].
The tests were conducted on specimens cut from the virgin products. Tests on the explant specimens were performed at a load at which run-out occurred for the former specimens, Figs. (6, 7).
3 . RESULTS
Twenty-one explants from different in-situ periods (2.5-6.0 month) were successfully harvested, but only 12 were of sufficient size for use in the fatigue tests. The structural integrity of explants harvested after 6 months in situ was very poor, making them mostly unsuitable for use in the fatigue test.
In the fatigue tests, the virgin products (9 segments) tested showed a Median Fatigue Limit (MFL) of 49 N ± 11 N and survived at 38 N (Fig. 5). At this load, all of the explant specimens tested failed (Fig. 6a). When testing specimens of different volume, the critical volume of a tested specimen has to be considered, since it has an influence on the strength. In our case, we selected specimens of similar dimensions and essentially identical critical volume in order to assure a direct comparability of the results. At 38 N, there was a moderate drop in fatigue life of the explant with increase in-situ time up to about 4 months, after which the drop was very steep (Fig. 6b). Fatigue test specimens fabricated from a point in an explant close to the L-shaped feature of the implant (that is, segments that were proximal to the osteotomy line, the area of maximum load in-situ) had a significantly shorter life than those fabricated from fragments taken farther from the osteotomy line (Fig. 7; p=0.0017). This trend is consistent with increase in stress as distance to osteotomy line decreases.
3.1 . Morphological Examinations
The surface of a virgin product was smooth, with traces of press flags arising from the melt joining performed as part of the manufacturing process (injection molding) (Fig. 8,a,b). On these surfaces, there were local cracks, which may have been caused by residual stresses during injection molding (Fig. 8c). Since these defects are under a flexural load in the vicinity of the neutral fiber, they most likely will not compromise the reliability of the implant,
The morphology of the fracture surface of fragments taken from an explanted implant varied with implant time in situ. After 4.5 months, with one exception, there was an absence of cracks running from the pores into the bulk of the implant (Fig. 9a). The exception is seen in the region close to the surface of the implant (i.e. close to the edge of the fracture surface: here, cavities were formed (Fig. 9b). After 9 mo, there were signs of severe internal degradation; the entire fracture surface shows cracks and pores which reach into the bulk of the implant (Fig. 9, c, d). This internal degradation resembles the surface-core degradation mechanism observed for “massive” poly(DL lactide) implants [26]. The development of the degradation features seen in the present explants agreed well the fatigue test results as well as the claims of the implant manufacturer that the mechanical strength of the implant is stable up to about 3 mo in situ [15]. The onset of the core degradation (as seen in Fig. 9b) will result in an initial decrease of the implant strength. A significant drop of the flexural strength will occur, if the internal degradation extends from the core of the implant (which does not contribute significantly to the strength due to its proximity to the neutral axis vicinity to the neutral fiber) to the implant surface. This occurs after in situ periods longer than 4 months. Fig. (10, a, b) illustrates in-vitro laboratory-aging associated surface roughness and dimples on the surface of the implants, also at bigger magnification these irregularities were contrasting the smooth surfaces of in-situ aged explants.
4 . DISCUSSION
PLDLLA-TMC has the advantage over previous copolymers to be more compliant and less brittle for an easier handling and more reliable clinical use (e.g. material has less tendency to break after only minute increase in torque as in other polymers, but the screws can be tightened with a slow long increase in torque until potential breakage). Moreover the 2.5 mm strength can be used in more complex fracture and osteotomy situations also. Yet the stability comes at the price of a large volume. Therefore degradation may be unreliable above all, as in-vitro laboratory, in-vivo animal studies cannot become transferred one-to-one to humans [18,19].
4.1 . Fatigue Tests
While all the virgin products in the present report showed run-out at 38N, all of the specimens fabricated from explants failed at this load level. For the explant specimens, although there was a trend of decreased failure load with increased in-situ time of the implants, this decrease was pronounced after 4 months in situ. Dry storage was chosen, as storage in aqueous medium would seriously accelerate degradation.
Several stability evaluations are published on the PLDLLA-6TMC material, which was evaluated within the present report. Nieminen et al. [15]. after subcutaneous implantation into sheep, explanted plates to find them to maintain their tensile strength for minimum 6 weeks. The maximum strength of the plates in 3-point bending tests diminishes gradually by 87% in 26 weeks. These results concord with our observations: up to 3-4 months in-situ, only a moderate fatigue strength reduction occurred (partially caused by in situ mechanical loading, partially caused by the onset of degradation); at 6 months in situ, severe loss of mechanical strength and significant internal degradation were observed. Although implants did not loose their shape after 6 months in-situ, fragmentation onset and increasing rate of material loss can be expected, which ultimately results in significant bulk degradation. The latter is a “skin-core-differentiation”, when degradation products accelerate degradation inside the implant, while surface material can become metabolized locally. This was observed in the scanning electron microscopy for the implants 9 months in-situ [17].
This present report affirmed other successful investigations on the mechanical performance of new and explanted resorbable implants [18,27]. Yet previous studies showed a tendency to higher recrystallization in in-situ aged versus in-vitro aged specimen (who by themselves were immersed in physiological buffer solution at 37°C). This was interpreted to be an effect of permanent intermittent loading. Therefore within the present study, a different approach was undertaken, determining the median fatigue limit of new implants with the staircase method. And as it was expected, explants showed a shorter fatigue life after three months in-situ compared with new implants.
Longer periods in-situ correlated with a shorter fatigue life and the effect became visible only after an implantation period of 3 months. This is an indication that initial retention strength is maintained during the first three critical months of bone healing. Resorption and mechanical loading during implantation, implantation period in-situ, and explantation will contribute to the loss of fatigue strength. Local variations of the fatigue strength of individual explants were observed, either due to initial variations of the materials strength or due to variations of the stresses in vivo. Initial variation in material strength was not found in the out-of-package samples therefore can be disregarded. In the clinical situation a complex mechanical loading of the implant occurs, which results in an inhomogeneous stress distribution. Close to the osteotomies and in the vicinity of specific geometric features of the implant (e.g. narrow connections or angular shape), stress concentrations will occur, which result in a higher probability of failure in these regions.
Buijs et al. [13,14]. investigate seven biodegradable and 2 titanium osteofixation systems. Plates and screws were fixed to 2 polymethylmethacrylate (PMMA) blocks to simulate bone segments. Plates and screws were subjected to tensile, side bending, and torsion tests. During tensile tests, strength of the osteofixation system was monitored; stiffness calculated for the tensile, side bending, and torsion tests. The 2 titanium systems (1.5 mm and 2.0 mm) are reported to evince significantly higher tensile strength and stiffness compared with 7 biodegradable systems (2.0 mm, 2.1 mm, and 2.5 mm). The 2.0 mm titanium system showed significantly higher side bending and torsion stiffness than the other 8 systems. It is concluded that the titanium osteofixation systems were (significantly) stronger and stiffer than the biodegradable systems. The BioSorb FX® (Linvatec Biomaterials Ltd, Tampere, Finland), LactoSorb® (Walter Lorenz Surgical Inc, Jacksonville, FL), and Inion CPS® 2.5 mm systems however show high mechanical device strength and stiffness. Resorb X® (KLS Martin GmbH & Co, Tuttlingen, Germany) and MacroPore® (MacroPore Biosurgery Inc, Memphis, TN) systems present as the least strong and stiff systems. A prospective evaluation could compare this present study’s findings with titanium plate explants and compare these to titanium out – of – package specimen. Minimal decrease of retention strength should be expected in titanium (not mentioning the unfortunate drawbacks of titanium plates) [28-35]. Vaananen et al. [11]. examine the hydrolytic stability of an ankle plate made from identical material over 12 weeks. A fracture of the lateral malleolus is simulated, and the parameters of cyclic loading chosen representing the physiological conditions during the healing period. Additionally, the effect of cyclic loading on degradation is investigated by measuring the inherent viscosity. In group I, the cyclic loading is conducted in four phases with gradual increments in estimated walking distance and speed during the healing period. In group II, cyclic loading is conducted after 12 weeks. Group III is used as a control for inherent viscosity measurements [18]. None of the specimens failed, no significant differences are found between the loaded groups in any of the parameters, no significant differences are found in inherent viscosities at 12 weeks. The initial fixation stability provided by the biodegradable ankle plate remains biomechanically unchanged over 12 weeks. Cyclic loading, applied either during, or after 12 weeks of hydrolytic degradation, does not appear having any clinically relevant effect on the fixation stability or the degradation properties. The present study confirmed flexural fatigue strength to only decrease after three months in-situ. However the in-vitro aging process cannot represent the in-situ (in-patient) situation. Although an elaborate approximation, loading does not correspond to the permanent intermittent complex loading that occurs in-situ. The loading patterns chosen by Vaananen [11]. are possibly representative for the loading situation of a plate used for fixation in the leg, but is certainly not applicable to the jaw, as permanent movement in swallowing of saliva and speaking occurs, even if no loading by hard food chewing occurs. Moreover the effect of enzymes, cell cytotoxicity and variation in loading are not sufficiently represented. The present report included these factors within the in-situ aging, as these factors cannot become reproduced in the laboratory.
4.2 . Degradation
Nieminen et al. [15] test the tissue reactions of the degrading identical PLDLLA-6TMC material. To the mandible and dorsal subcutis of 12 sheep, plates and screws are implanted. The animals are sacrificed at 6-156 weeks, paraffin and methylmetacrylate techniques provide histologies. During follow-up, degradative and mechanical properties are measured of in-vivo and in-vitro implants. Upon light microscopy, in-vivo implant material begins to fragment at 52 weeks and cannot be detected at 104 weeks, which is at a point in time far later than the retrieval of the implants, studied in this work. Identical polymer implants have different patterns of resorption depending on e.g. local loading, perfusion, motion, temperature distribution that largely differs between dorsal subcutis and the craniofacial bones as between sheep and humans [19,22]. This makes specific study of a certain polymer a necessity depending on these named factors as has been performed with this study.
We investigated fracture surfaces of explants by SEM, which fractured during the explantation process and detected severe signs of internal degradation after 6 months in-situ. This observation, however, gives little information on the time period in-situ, when a macroscopic fragmentation of the implants will occur. Nieminen et al. [15] see no significant foreign body reactions in the mandibles, which were confirmed by our patients, who within clinical use were n=80 receiving PLDLLA-TMC material, when none developed foreign body reactions that were clinically apparent. The dorsal subcutis of the sheep examined by Nieminen et al. [15]. disclosed mild reactions, which are, however, not of clinical significance. The implants in vitro maintain their entire mass for 26 weeks and lose 63-80% of the mass by week 104. Inherent viscosity of the implants in vitro and in vivo diminishes uniformly, screws retain their shear strength for 12-16 weeks. Many more studies regarding the hydrolysis of older poly(L-lactide-co-polyglycolide) (PLGA) copolymer chains in-vitro to degradable low-molecular-weight units, through water uptake into the implant body are to be found in the literature. In-vivo but notin-situ in chinchilla rabbit femurs, PLGA complete degradation required 12 months [29,36,37]; in parietal craniotomies of rabbits 12 months [20]; and in minipigs 18 months. [10]. Differences in resorption times are probably due to different resorption mechanisms, depending on the species of laboratory animals. Furthermore, implant size, shape, molecular weight, composition, monomer conversion, macromolecular orientation and implant position in the body [19]. make in-patient location-specific assessment necessary. The combination of the copolymers PLLA for adequate strength and the less stable poly DL-lactic-acid (PDLLA) for fast, reliable degradation as poly(L-lactide-co-DL-lactide) (P(L/DL)LA) constitute together with PLGA available and clinically applied resorbable miniplate osteosyntheses today. Addition of trimethylenecarbonate to P(L/DL)LA –TMC provides higher elasticity i.e. a polymer that is less brittle as pure PLLA or P(L/DL)LA, which is advantageous in clinical handling [38].
Losken et al. [39] examine the degradation within 1 year and foreign body reaction and cyst formation evaluating the rate of similar biodegradation of “Inion CPS Baby” ® biodegradable plates and screws under different clinical circumstances in the rabbit craniofacial skeleton and evaluate their efficacy for use in pediatric craniofacial surgery. In these implants DL-PLLA has been replaced by PGA maintaining a similar TMC fraction, therefore direct comparison with the results of the present report is not possible. These implants are tested in a rabbit model: plates are applied to the frontal bone, over a bony defect of the parietal bone, to a nasal bone fracture, and inserted in the subcutaneous space over occipital bone in thirty 6-week-old rabbits. At 9 months, the plates and screws have effectively biodegraded no longer have retention power, which however is difficult to evaluate, as the cooking of paraffin embedding washes out residual poly-lactide. However fragmentation of the implant material was noted. Residual implant material was still present on gross and histological examination in rabbits at 9, 12, 15, and 18 months. Residue of a screw was still palpable in 1 rabbit at 18 months. There was no evidence of cyst formation in any of the examined specimens. Macrophages and giant cells were present in most specimens. The authors document a relatively short resorption time of (9 months) and normal inflammatory sequelae in an adult rabbit model and suggest that these plates may be used safely in fixing the pediatric craniofacial skeleton. A similar study could be performed with the INION CPS® 2.0 and 2.5mm system material.
SEM images, acquired to support the evidence found in the mean fatigue strength showed marks of the production process; at 4 ½ months in-situ, slight effects of the degradation became visible and at 9 months in situ effects of degradation became clearly visible.
P(L/DL)LA examined earlier as well as the PLDLLA-6TMC implants are amorphous, despite the fact that the raw material granules have a crystallinity of around 10%. In PLLA, PLDLLA-6TMC and PLGA, hydrolysis precedes phagocytosis [7,9,40]. This should obviate bulky degradation and the accumulation of osmotically active polymer debris that induces local osteolysis in adjacent bone, which is beyond the absorptive capacity of the surrounding tissues. [7,9,40]. When chinchilla rabbit bone was examined under a light microscope, P(L/DL)LA implants were reported to have degraded fully and disappeared after 12 months [36]. Studies using sheep found no discharge or swelling after the 6-month follow-up [41].
In-vitro degradation, simulating spontaneous hydrolysis, cannot be compared to the in-vivo situation, the microclimate in which enzymatic degradation, recrystallization and phagocytosis occur, cannot be simulated by in-vitro studies and this has been confirmed with this study’s unsuccessful assay of in-vitro aging. In addition, animal models cannot be compared, as in humans hydrolytic degradation predominates, while in animals (such as rabbits) cellular enzymatic processes play a more prominent role [36]. Interindividual degradation variability in patients and individual risk factors are not currently known with certainty. This study assesses, whether clinical in-patient degradation is reliable by fatigue testing and not inherent viscosity or cristallinity, which has been proven before for very similar polymers [18].
Differences in resorbable polymer osteofixations have been reported to result from several factors: their shape, the composition of the material used, molecular weight, monomer conversion, macromolecular orientation and the different animal models and body regions in which they are used [19,36]. Therefore the in-situ results were compared with the in-vitro results [17,18]. Clinical in-patient studies usually do not provide sufficiently long follow-up periods, and do not sufficiently consider side effects, reossification and palpability [4]. Also, these studies do not emphasize what really happens to the material in-situ, but instead draw conclusions based on indirect signs of degradation. For example when in animals-studies, polymers had completely resorbed and were not visible under light microscope, bur holes had yet not completely reossified. Radiological reossification, often used as clinical in-patient marker of degradation, may therefore be captious when determining complete resorption [36]. Until now, no degradation studies had been carried out in-patients to evaluate degradation kinetics and reliability directly from PLDLLA-6TMC explants as was done in the present study. Prior to the present study, degradation studies had not used explants to evaluate degradation kinetics and the reliability of decomposition in patients.
4.3 . Clinical Consequences and Limitations
Laughlin et al. [16] clinically test resorbable plates with the identical polymer tested here versus titanium 2-mm plates regarding fracture healing, bone union and function. Fifty mandibular fractures are included being either mandibular body, symphysis, angle, or ramus fractures, requiring open reduction internal fixation. Data are compared with literature norms for titanium and nonrigid fixation data from a prospective study performed within the same institution. Clinical and radiographic evaluation indicated bone union at the eighth follow-up visit, 3(6%) sites had clinical signs of infection treated immediately upon presentation, with fracture union by 8 weeks. No revision surgery was required; 12 screw heads fractured during insertion, immediately replaced without significant fracture sequelae.
Vaananen et al. [12] develop a "free-form" osteofixation plate, equipped with pilot holes. The plate construction allows screw placement in optimal position. The screw heads are either countersunk into the plate or cut away. The plate further can be cut and contoured to match the bone. The mechanical properties of the freeform osteofixation plate made from the identical polymer are compared to the conventional biodegradable plate. Acrylic pipes fixed together with plates and screws are tested with tensile and cantilever bending to measure the fixation properties. The tensile test loads the samples with constant 5 mm/min speed until failure of fixation. Yield load, maximum failure load, and initial stiffness are recorded, and the failure mode visually determined. In cantilever bending test, samples are loaded with a constant speed of 50 mm/min (with a moment arm of 45mm) until failure of fixation. The yield bending moment and initial stiffness are recorded, and the failure mode determined. The results show the new free-form plate to provide fixation at least as strong as the tested conventional biodegradable plate. No clinically relevant difference is found between free-form plate fixation with countersunk screws and fixation with screws without heads.
A larger case number is planned in future evaluations. However, within a technology to avoid secondary procedures, this will require considerable time. Only large-scale long-term clinical research will ultimately show the optimum clinical biocompatibility and physico-chemical characteristics of biodegradable orthopedic implants [4].
CONFLICT OF INTEREST
None declared.
ACKNOWLEDGEMENT
Acknowledgement of Funding: none for this evaluation